If we’re going to use rocks and fossils to learn about Earth’s history, then we need a way to know how old they are. Scientists have two major ways of learning about the ages of rocks and fossils:
- Relative dating means studying rocks and fossils to learn which ones are older and which are younger. Note that, by itself, relative dating does not tell us the precise age of any rock or fossil, but only whether it is younger or older than other rocks and fossils.
- Absolute dating means finding the actual ages of rocks or fossils; that is, the number of years since they formed. For a rock, this means how long it has been since the rock took on its current form; for example, the age of an igneous rock is the time since it solidified from molten rock. For a fossil, this means knowing how long it has been since the organism that left the fossil died.
Absolute dating is clearly preferable, because it can give us a very precise timeline. However, it is not always possible (for reasons we’ll discuss shortly), so scientists generally use both methods together. Moreover, the primary method of absolute dating was only discovered a little over a century ago. Before that, geologists could generally determine only relative ages, not absolute ages.
Discussion
Relative and Absolute Family Tree
The figure below shows a generic “biological family tree” that covers five generations (for example, from you to your great-great-grandparents). Assume that this is the family tree of someone that you don’t know personally. Discuss the following questions to help you understand the difference between relative and absolute ages.
- Can you be certain that great-great-grandmother 1 is older than great-grandmother 1? Why or why not?
- Can you be certain that great-great-grandmother 1 is older than great-grandmother 3? Come up with an example of ages at which different generations gave birth to explain your answer.
- In general terms, describe all the relative age relationships that you can establish from the family tree.
- Describe a few different kinds of evidence that would allow you to convert the relative age relationships into absolute ages for some or all of the people in the family tree.
- Suppose you find two photos of high school graduations. The first shows great-great-grandmother 1 and says “class of 1935.” The second shows grandmother 1 and says “class of 1975.” What does this tell you about the age of great-grandmother 1? Does it tell you about anyone else’s age? Explain.
The concept of relative versus absolute ages may at first be confusing to students, but it is not difficult to understand. This discussion is designed to help. Notes on answers.
- (1) Yes, because great-great-grandmother 1 is the mother of great-grandmother 1, so she must be older.
- (2) No, because great-great-grandmother 1 is on a different branch of the tree from great-grandmother 3. For the example, there are many possible ways to do it, but one of the simplest would be to use different time between generations on the two relevant branches. E.g.:
- If you assume 20 years between generations on the branch leading to great-great-grandmother 1, then she was born 4 x 20 = 80 years before “me.”
- If you assume 30 years between generations on the branch leading to great-grandmother 3, then she was born 3 x 30 = 90 years before “me” — which makes her older than great-great-grandmother 1, even though she is not as far back on the family tree.
- (3) Along any branch, you know that each preceding generation must be older than the one that follows.
- (4) You need evidence that gives particular dates, such as dated photos, birth certificates, etc.
- (5) Assuming that high school graduation occurs around age 18, the graduation photos tell you that great-great-grandmother 1 was born around 1917 and grandmother 1 around 1957. This means that great-grandmother 1 must have been born between 1917 and 1957. We can narrow this range further by applying what we know about ages of motherhood. For example, if we assume that the women on this family tree did not give birth before graduating from high school at age 18, then great-grandmother 1 had to have been born at least about 18 years after 1917 and 18 years before 1957, which narrows her likely birth year to being between 1935 and 1945. In a similar way, we know that mother 1 was born after 1957 and most likely after about 1975.
Note: These ideas in which knowing some ages put constraints on others are the idea we will discuss shortly as “bracketing” in the geological record.
Relative Dating
Let’s start by considering relative dating, which is not only more common but is something that you can often do yourself, without needing any fancy scientific equipment. The reason you can do this is because the basic principles of relative dating are all pretty obvious, at least once you think about them. We’ll focus on five major principles.
Relative Ages from Rock Strata
The first two principles apply to layers of rock, which you’ll remember are also called strata . We’ve discussed these first two principles already, but let’s restate them to make sure they are clear:
Relative dating principle 1: Younger rocks form on top of older ones . Therefore, we can generally assume that deeper strata are older than the strata that lie above them. You can see this idea in the Grand Canyon photo that opened this chapter.
In some cases, geological forces such as uplift have tilted or folded the strata after they formed, so that they are no longer horizontal (Figure 5.1.3–1). In these cases, geologists must carefully study the strata to figure out which layers were laid down first .
Relative dating principle 2: We can use fossils to compare rock strata from different locations: Strata that contain fossils of the same types must be approximately the same age . Review Figure 5.1.2–3, where we already showed how this idea works.
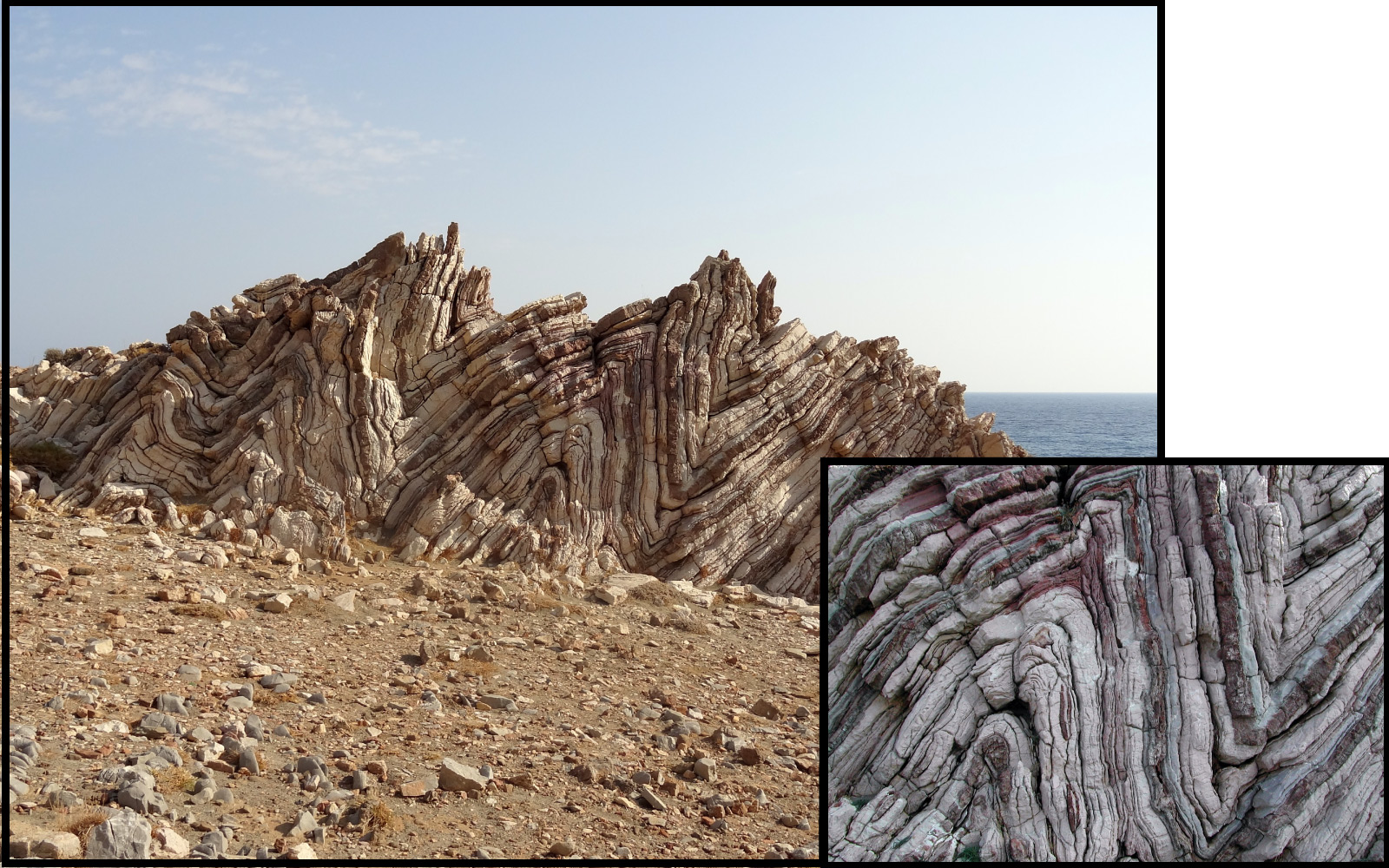
Inclusions (of one rock within another)
The first two principles allow us to figure out which layers are younger or older in a general way. However, rocks and strata can be more complicated in their details, because in some cases rocks of different ages can be mixed together.
Sometimes, a piece of old rock can become enclosed within younger rock. Because this means that the older rock has become “included in” the younger rock, geologists refer to the older rocks as inclusions .
Figure 5.1.3–2 shows two examples of inclusions. The first case (Figure 5.1.3–2a) shows an older igneous rock that is enclosed within a younger one. The second case (Figure 5.1.3–2b) shows that a sedimentary rock layer is composed of many individual rocks or grains; these rocks and grains must be older than the layer itself . Use the questions that follow the figure to make sure you understand these ideas.
Figure 5.1.3–2 – Older rocks can become inclusions in younger rocks, meaning that the pieces of older rock have become surrounded by (or “included in”) younger rock.
Discuss the following questions with a classmate. Then click to open the answers to see if they agree with what you came up with.
- Consider Figure 5.1.3–2a, in which you see a dark-colored igneous rock enclosed within lighter-colored igneous rock. When we say that the dark-colored rock is “older,” what do we mean?
- Knowing that both the dark and light rock in Figure 5.1.3–2a are igneous, what can you conclude how the dark rock became enclosed by the light rock?
The dark, older rock must have become surrounded by molten rock, and this molten rock became the light-colored rock when it cooled and solidified. We can also conclude that the dark rock itself did not melt, even as it became surrounded by the molten light-colored rock. (Because if it did, the two sets of molten rock would have gotten mixed together, in which case we would not see a clear boundary between the two colors.)
- Explain why what we see in Figure 5.1.3–2a tells us the relative ages of the dark- and light-colored rock, but not the absolute ages.
As we’ve already discussed, the fact that the dark-colored rock is enclosed by the light-colored rock tells us that the dark-colored rock is older. This means we know their relative ages. However, this alone does not give us any information about their absolute ages. That is, we still don’t know exactly when either piece of rock formed, nor do we know whether the darker rock formed thousands, millions, or even billions of years before the light-colored rock.
- Now consider Figure 5.1.3–2b. How can you be sure that the small rocks within this sedimentary layer are older than the layer itself?
Our third principle summarizes the above ideas:
Relative dating principle 3: When one rock is enclosed within another, the enclosed rock (the “inclusion”) must be older than the rock that surrounds it.
Claim-Evidence-Reasoning Activity
Rocks and Fossils in Sedimentary Strata
Based on the ideas of relative dating that we have discussed so far, use evidence and reasoning to support or refute the following two claims about sedimentary strata (rock layers).
Claim 1: The smaller rocks or grains found in a sedimentary rock layer must be older than the layer itself.
Claim 2: Fossils found in a sedimentary rock layer are about the same age as the layer itself.
This CER activity will help check that students are understanding the basic principles of relative dating described so far. Both claims are true. Notes:
- Claim 1: Students should be able to use the ideas discussed and shown in Figure 5.1.3–2 to support the claim.
- Claim 2: For this claim, students should be able to think back to how fossils form when organisms die and become buried in sediments, and use this fact to show that fossils must reside in the layer in which they became embedded.
Cross-cutting
Our fourth principle for learning about relative ages involves patterns that cut through rocks, which are called cross-cutting patterns because they tell us that something has “cut across” a rock. Consider, for example, the crack visible in Figure 5.1.3–2a. Because this crack cuts through both the older enclosed rock and the younger surrounding rock, the crack must have formed after both of the rocks formed. In other words, a crack must always be younger than the rock that it cuts through.
Figure 5.1.3–3 shows another common example of a cross-cutting pattern. In this case, the larger rock has been cut through by a different type of rock to make a series of dikes , which get their name by analogy to the dikes that people build out of earth to prevent flooding. In rock, dikes typically look like long, narrow tubes or like sets of veins (like the veins in your body). Dikes form when molten rock flows into a crack in an existing rock, filling in and sometimes widening (or even creating) the crack. This means that, like a crack, the rock making up the dike must be younger than the surrounding rock, since the surrounding rock had to have already been there before the molten rock came through. We can now state our fourth principle:
Relative dating principle 4: Anything that cuts through a rock — such as a crack or a dike made up of other rock — must be younger than the rock that it cuts through.
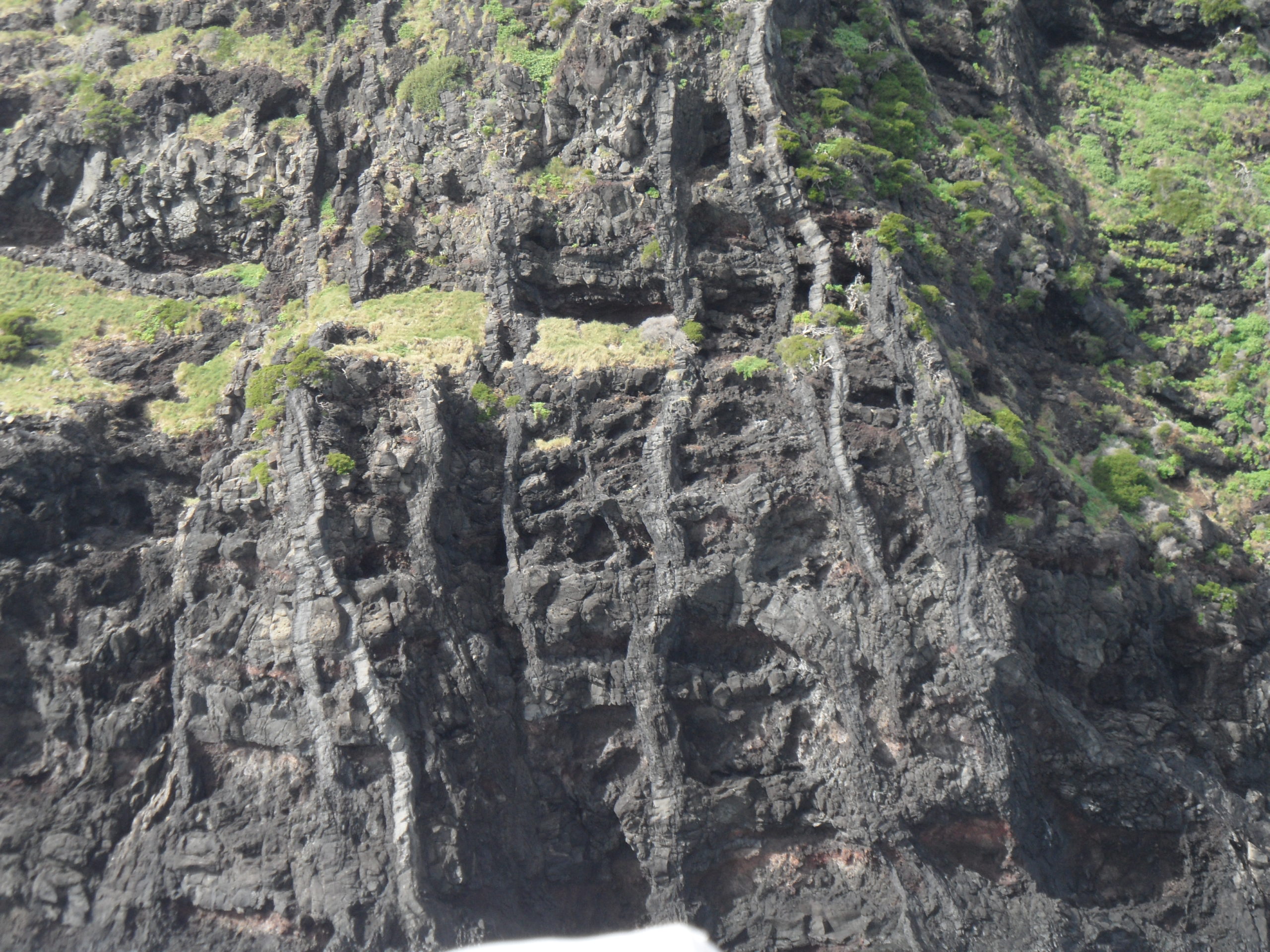
Activity/Demonstration
Dike Formation
We can make a model using wax, sand, and water to show one way in which dikes form. The video below shows the model in action, or your teacher may do a demonstration of the model. Note that the model has three major components
- The bottom layer is wax. In this case, the wax represents rock deep underground.
- Above the wax is a layer of sand, representing a buried layer of sedimentary rock.
- The top layer is water, which in this case represents rock that is above the sedimentary rock.
To illustrate the process of dike formation, the wax is heated at the bottom, so that it begins to melt (representing molten rock). Observe what happens, then discuss the following questions.
- Why do you think the melted wax rises upward (rather than, say, staying in place or sinking)? How do you think this is similar to what happens with molten rock underground?
- Why do you think the wax rises upward in narrow “tubes” rather than all across the bottom?
- Suppose we could suddenly cool the entire model just after the first tubes begin to form (before any wax breaks through the water surface). Explain how this would leave dikes of wax in the sand representing the sedimentary rock.
- Explain how, at the end (when the wax has risen through to the surface), this model represents a volcanic eruption.
Credit: Royal Society of Chemistry
This activity will help students understand how dikes form, and is based on a standard demonstration called a “wax volcano.” You can simply have students watch the included video, but if you have the needed equipment, it will be more fun if you do the demonstration yourself in class, following the instructions given here. Notes on the discussion questions:
- The wax rises upward because melted wax has lower density than solid wax. The same is true for rock: molten rock is less dense than solid rock, which gives it a tendency to rise upward. (In fact, almost all materials are less dense in liquid form than in solid form, with water as a notable exception since solid ice is less dense than liquid water (which is why ice floats).)
- There are multiple possible explanations for the tubes, but two of the most important are: (1) the heating is unlikely to be perfectly uniform, so that some spots melt sooner than others; and (2) the sand may have “weak spots” where wax can begin to rise up more easily. In both cases, the initial factors tend to accelerate, so that most of the melted wax is channeled up in these tubes rather than all across the bottom.
- If we suddenly cooled the model, the wax “tubes” would become “frozen” into the sand, just as dikes of igneous rock can become frozen into sedimentary rock.
- Once the was breaks through the surface, it flows outward across the surface, just as molten lava flows outward from a volcanic eruption.
Activity
Relative Rock Ages
Figure 5.1.3–4 below is photo of a rock that clearly contains at least four components, labeled A through D in the key to the right:
[A] A set of tan-colored "dikes" (shaped like a set of veins).
[B] A very dark colored rock.
[C] A few light-grey lines of rock extending horizontally.
[D] A set of nearly vertical and very narrow "scratch marks" on the rock.
Working in small groups, use what you’ve learned above to determine the relative ages of the four components. That is, determine which is the oldest, second-oldest, third-oldest, and youngest.
This activity has students work together to see if they can identify the relative age relationships, which are as follows:
- Oldest: (B), because all the other features cross through this dark-colored rock.
- Second-oldest: (C), because these light-grey rock lines cut through the oldest component, but not through the tan-colored component (A).
- Third-oldest: (A), because these tan dikes cut through both components (B) and (C).
- Youngest: (D), because these scratch marks go across all of the other components.
Fyi: This activity and rock photo are based on Exercise 8.1 found here. As the original source notes, this rock is from Horseshoe Bay, B.C. The oldest component (B) is a metamorphic basalt. The light-grey dikes (C) are igneous rock that forced its way into the metamorphic rock. The tan dikes (A) are also igneous, forced through at a later date. The “scratch marks” (D) are a remnant of the blasting (by humans) that exposed the rock.
“Unconformities”
When you look at the layering in the Grand Canyon, you might at first think you are seeing a continuous record of Earth’s history, with layer upon layer piled up steadily over millions of years. However, if you look more closely, you’ll start to notice that there are some clear breaks in the record.
Figure 5.20 shows an example. The dashed line represents a place in the Grand Canyon where a lower layer of tilted rocks runs into an upper layer of horizontal rocks, which clearly indicates some type of break in the geological record that is much more significant that what we see when layers are neatly stacked. This type of sharp division between rock layers is called an unconformity , and it must represent some major distinction between the two layers it separates. In this case, absolute dating (which we’ll discuss shortly) tells geologists that the rocks just below the dashed line are hundreds of millions of years older than the rocks just above it . This tells geologists that, at this location in the Grand Canyon, a big chunk of Earth’s rock record has gone missing.
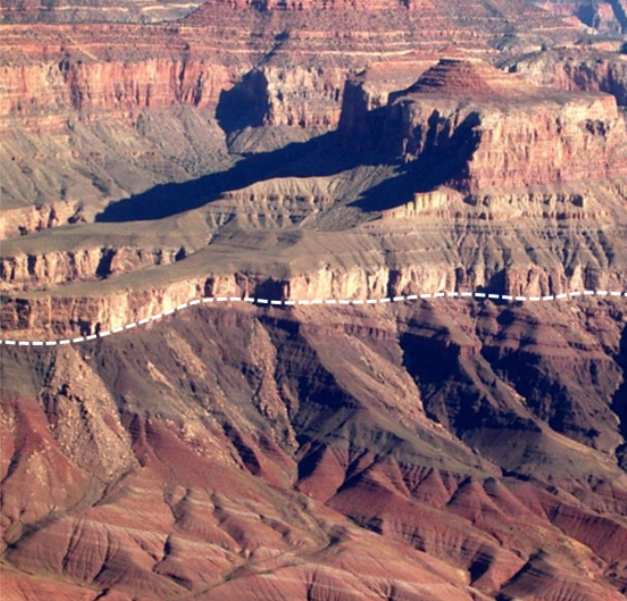
What could have happened to create such a large gap in the rock record? There are two general possibilities (Figure 5.1.3–6). First, as shown in Figure 5.1.3–6a, a gap (marked by the unconformity) can occur if, for some reason, deposition stopped for millions of years before resuming. In this case, the “missing” part of the rock record was never there in the first place. Second, as shown in Figure 5.1.3–6b, the “missing layers” might once have existed, but they were eroded away. This gives us our fifth and final principle of relative dating:
Relative dating principle 5: A clear break between rock layers, called an unconformity , marks a place where part of the rock record is missing, so that the rocks just below the unconformity formed millions of years before the rocks just above it.
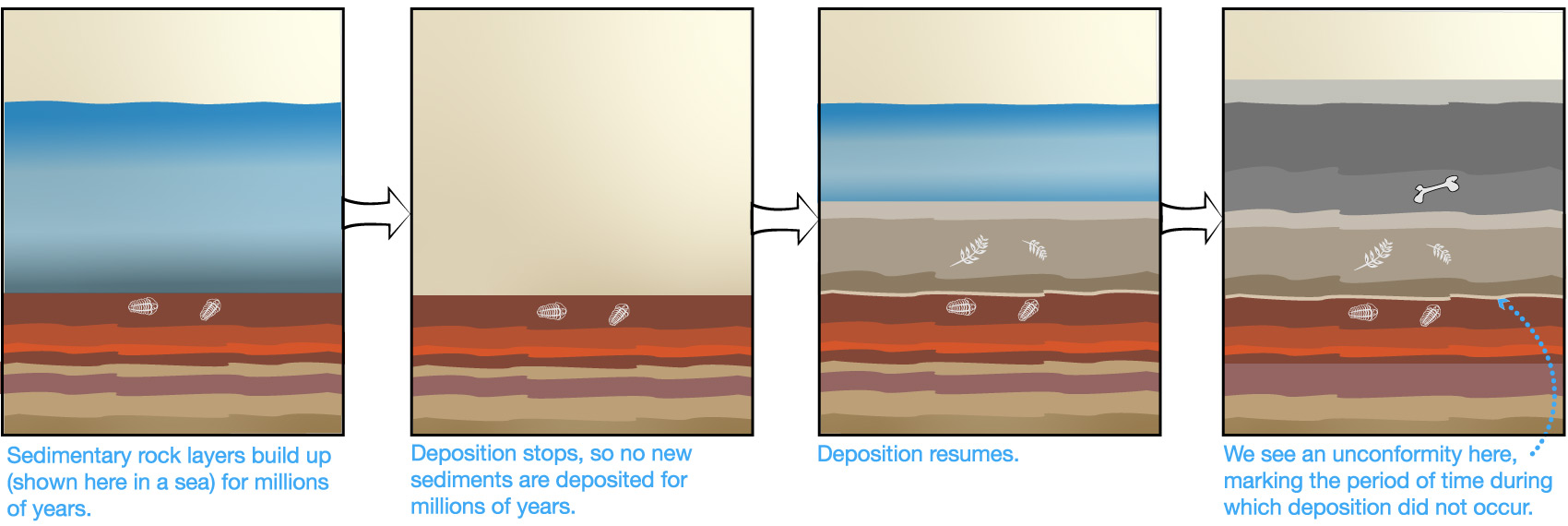
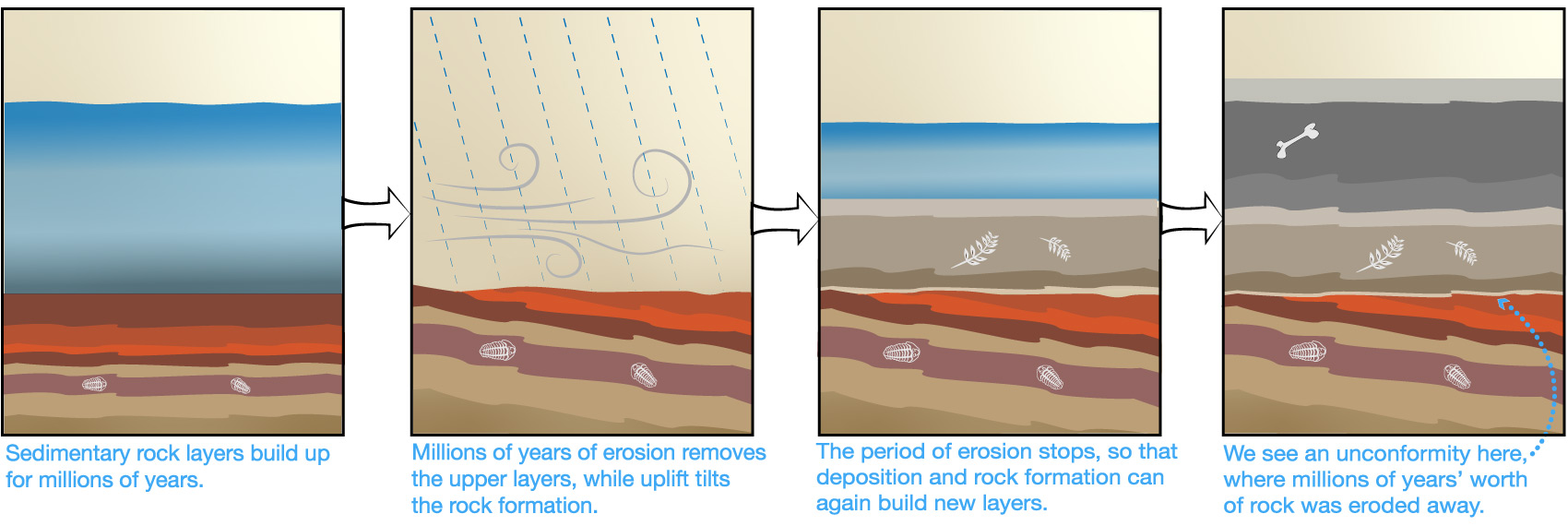
Figure 5.1.3–6 – This illustration shows two general ways in which part of the rock record can be “missing” from rock strata, causing us to see an unconformity. In many or most cases, some combination of both things may have happened. Credit: Zofostro Science.
Key Concepts: Relative Dating Principles
Five major principles are used to figure out the relative ages — meaning which are older and which are younger — of rocks (and fossils found within the rocks).
- Younger rocks form on top of older ones, so that deeper strata are generally older than the strata that lie above them.
- Strata that contain fossils of the same types must be approximately the same ages.
- When one rock is enclosed within another, the enclosed rock (the “inclusion”) must be older than the rock that surrounds it.
- Anything that cuts through a rock — such as a crack or a dike made up of other rock — must be younger than the rock that it cuts through.
- A clear break between rock layers, called an unconformity, marks a place where part of the rock record is missing, so that the rocks just below the unconformity formed millions of years before the rocks just above it.
As an example of how all these ideas come together, Figure 5.1.3–7 repeats the chapter opening photo of the Grand Canyon, and next to it adds a chart that shows the ages (from absolute dating techniques) and depths of various rock layers. The key at the top shows the names geologists give to the various layers. (You do not need to learn any of these names.) Note that the photo in Figure 5.1.3–7 doesn’t clearly show the tilted layers of the “Grand Canyon Supergroup Rocks,” but you can see these below the dashed line in Figure 5.1.3–5.
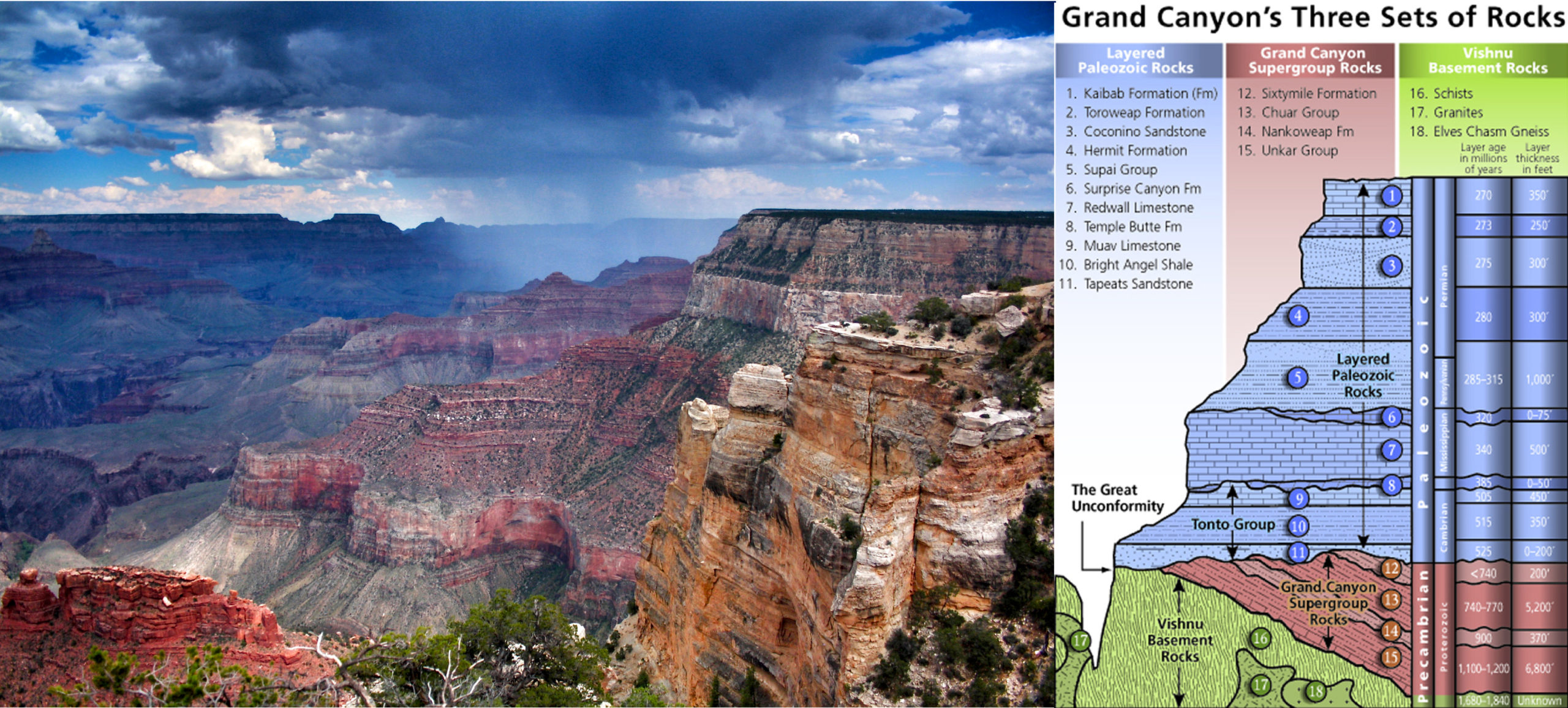
Activity
Grand Canyon Time Line
Study the chart in Figure 5.1.3–7, and do the following.
- To make sure you understand the chart, work in small groups to identify correspondences between the layers identified on the chart and what you see in the photo of the Grand Canyon wall in that figure and in Figure 5.1.3–5.
- Use the timeline you made in Chapter 4. Along the timeline, add labels for the top layer of the Grand Canyon (the “Kaibab Formation,” which is layer 1 in the chart) the bottom layer (the “Vishnu Basement Rocks”), and at least two other layers found in between.
Wow Factor
Visit the Grand Canyon
Coming Soon . . .
Absolute Dating
Relative dating is very useful even by itself. For example, relative dating techniques helped scientists to establish basic principles of geology and to determine the pattern of evolution that has occurred in the past. But relative dating is even more useful if we can assign a scale to it by learning the precise age of a particular rock, rock layer, or fossil. This is the goal of absolute dating.
For relatively recent objects, there are many ways to establish absolute dates. For example, some archeological artifacts (meaning objects made by humans) have dates written on them, allowing archaeologists to know exactly when they were made. However, because writing is only a few thousand years old, this method of absolute dating is possible only for certain objects made during this recent period of human history. Scientists can go somewhat further back in time for wood artifacts, because tree rings can tell us the age of a tree. By comparing living trees and wood from dead trees, scientists can in some cases use tree rings for absolute dating going back as far as about 25,000 years ago. Of course, this is only possible when we find wood, and it is still extremely limited, because 25,000 years is such a tiny fraction of Earth’s overall history.
Discussion
Tree Rings and Perspective on Time
We’ve used several different methods of trying to understand geological time, including making a time line for Earth’s history. Here, let’s do one that requires only a simple calculation. Working individually or in pairs, fill in the blank in the following statement:
Scientists can at best use tree rings to determine the age of wood artifacts from about 25,000 years ago. Earth itself is ______ times as old as these artifacts.
This is a simple math exercise designed to give perspective on time. Since Earth is about 4.5 billion years old, dividing by 25,000 years tells us that Earth is 180,000 times older than the artifacts. Notes:
- Students could of course do the division on a calculator, but encourage them to instead use “mental math.” In this case, the mental math procedure might look like:
- If students remember that 1 million is 1,000 times larger than 1 thousand, then they can immediately recognize that 25 million years is 1,000 times longer than 25,000 years.
- Remembering that four quarters make a dollar, students can then realize that 100 million years is 4 times as long as 25 million years, which means it is 4,000 times as long as 25,000 years.
- Next, recognize that 1 billion years is 10 times as long as 100 million years, which means it is 10 x 4,000 = 40,000 times as long as 25,000 years.
- Finally, Earth’s 4.5-billion-year age means the final answer is 4.5 times what we’ve found so far for 1 billion years. The “mental math” way to do this part is start by realizing 4 billion years must therefore be 4 x 40,000 = 160,000 times as long as 25,000 years, while 0.5 billion years is 0.5 x 40,000 = 20,000 times as long. Putting these together, the 4.5-billion-year age of Earth is 160,0000 + 20,000 = 180,000 times as long as 25,000 years.
- Once students get the answer, be sure to take a little time to consider the perspective built. That is, even though 25,000 years sounds like an incredibility long time to us, Earth itself is 180,000 times as old.
Radiometric Dating
Determining the ages of rocks and fossils requires methods that can go back much farther than tree rings. The best and most accurate method of doing this is through something that scientists call radiometric dating . This method is based on careful study of particular atoms that are said to be radioactive , which in science simply means that they tend to change or break apart, thereby turning the original atoms into different atoms. Radioactivity always changes atoms in a steady, predictable way. For example, uranium atoms are always radioactive in a way that very gradually turns them into atoms of lead — so gradually that about half the uranium atoms that Earth was born with have not yet changed at all.
Connections—Etymology
Radioactive
Today, the word radioactive has become common in everyday speech. It is usually used to describe something that is dangerous or that you would want to stay away from. For example, people might say that a disgraced politician has “become radioactive,” meaning that no one wants to associate with him anymore. However, the original meaning of the word was purely scientific.
In fact, the words radioactive and radioactivity were invented by the famous scientist Marie Curie (1867–1934), who was the first woman ever to be awarded a Nobel Prize and the first person ever to receive two Nobel Prizes (one in physics and one in chemistry). She invented these words for a simple reason: Her work proved that x-rays that had been observed to come from uranium-containing minerals were coming from the uranium atoms themselves. She therefore concluded that the uranium atoms were “active” in producing the “rays” or “radiation,” so she put the two words together to say that the atoms were “radioactive.” She and other scientists later learned that radioactivity is the result of changes that occur in the nuclei of some atoms, so we now use the term radioactive to refer to any atom that can spontaneously undergo these types of changes.
This steady, predicable change in radioactive materials is the basis of radiometric dating. You can see how it works with uranium: Because scientists have measured the rate at which uranium gradually turns into lead, careful measurement of the amounts of uranium and lead that are mixed together in a rock can tell us the rock’s age (Figure 5.1.3–8).
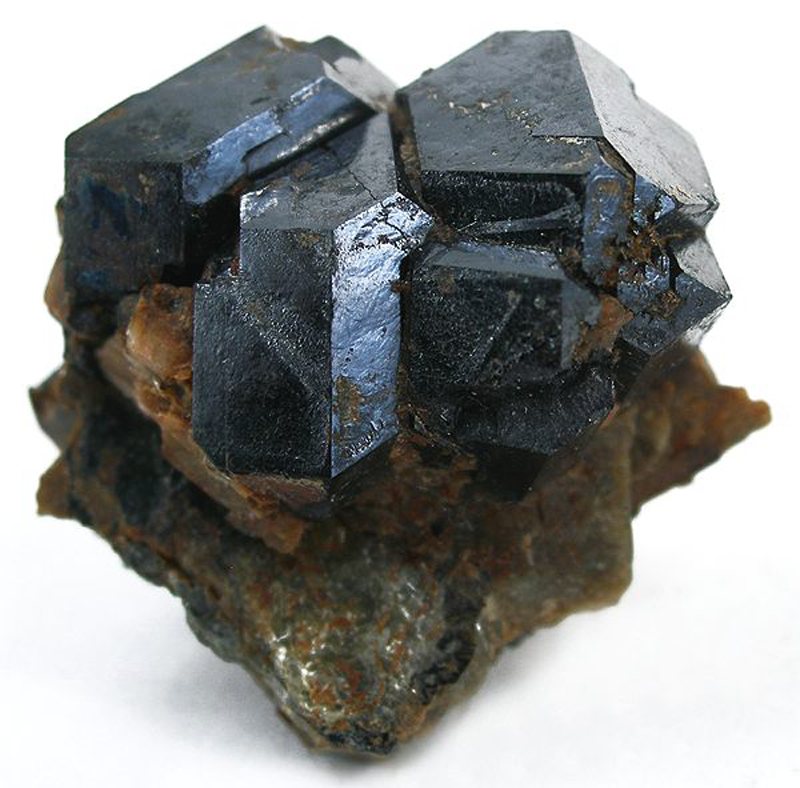
Figure 5.1.3–8 – This rock contains large crystals of the mineral uraninite (also called “pitchblende”),
which is rich in uranium. Because uranium atoms turn into lead atoms in a gradual, predictable way,
careful measurement of the amounts of uranium and lead mixed together in the crystals can tell
scientists the age of this rock. Credit: Robert Lavinsky.
Uranium is only one of many different radioactive materials, each of which undergoes changes in its own steady, predictable way. By now, scientists have carefully measured the rates at which these changes occur for many different radioactive materials, so all of these materials can be used for radiometric dating of rocks and fossils. This makes radiometric dating the most precise and most reliable method for the absolute dating of rocks and fossils. If you’d like to understand this process in more detail, read the Take it to the Next Level box on radiometric dating below.
Dating Rocks and Fossils
Radiometric dating can work so well that you might wonder why scientists would ever use anything else. The reason is that radiometric dating is not always possible. More specifically, it is possible only for rocks or fossils that meet three conditions:
- The rock or fossil must contain radioactive material, since that is the material that is used in radiometric dating.
- The radioactive atoms (and/or the atoms they turn into) must still be in their original places within the rock or fossil. This is because radiometric dating is based on measuring how many of the radioactive atoms have already changed into another type of atom. Therefore, if the atoms have been rearranged in any significant way (such as if the rock has melted), then we cannot get a reliable age.
- The rock or fossil that is used for radiometric dating must have a single age. For example, imagine that we try to date a rock like the one in Figure 5.1.3–2a, in which an older rock is enclosed within a younger rock. Because radiometric dating uses only a small sample of a rock, we will get different ages depending on whether we take the sample from the older portion or the younger portion .
Although many people are surprised to learn this, the first condition is easy to meet, because almost all rocks and minerals contain at least small amounts of radioactive material. This means that a low-level of natural radioactivity is all around us, but the level is low enough that it is not harmful.
The second and third conditions are more challenging. Use the following questions to think about what they mean for radiometric dating of different types of rock and for fossils.
Discuss the following questions with a classmate. Then click to open the answers to see if they agree with what you came up with.
- For igneous rocks, would you expect radiometric dating to be: (a) almost always possible; (b) sometimes possible; or (c) rarely possible? Explain.
- For metamorphic rocks, would you expect radiometric dating to be: (a) almost always possible; (b) sometimes possible; or (c) rarely possible? Explain.
- For sedimentary rocks, would you expect radiometric dating to be: (a) almost always possible; (b) sometimes possible; or (c) rarely possible? Explain.
Radiometric dating is rarely possible for sedimentary rocks, because sedimentary rocks are made from older rocks and grains that became cemented together. This means that if you do radiometric dating with sedimentary rocks, you are actually finding the ages of the older rocks or grains that make up the sedimentary rock (each of which may have a different age), not of the sedimentary rocks themselves.
- For fossils, would you expect radiometric dating to be: (a) almost always possible; (b) sometimes possible; or (c) rarely possible? Explain.
Radiometric dating is rarely possible for fossils. To understand why, remember that most fossils do not contain the actual remains of any of the original living matter . Instead, the once-living matter has been replaced with minerals, which means that even if you get a date from minerals in the fossil, it won’t be the date at which the organism originally died.
Age Bracketing
Given the difficulties of radiometric dating for sedimentary rocks or fossils, you might wonder how scientists learn absolute ages in these cases. The answer is that scientists look for clues that can tell us the minimum and maximum possible ages of a sedimentary rock layer and of any fossils within that layer. The range from the minimum to the maximum possible age is sometimes called an age “bracket,” so this process is called bracketing .
Discussion
Bracketing in the Family Tree
Look again at the biological family tree you discussed earlier. Recall that in question 5 of the earlier discussion, you had found two high school graduation photos: The first showed great-great-grandmother in the class of 1935 and the second showed grandmother 1 in the class of 1975. Explain again what this allowed you to conclude about the age of great-grandmother 1, and then explain how this is an example of “bracketing” similar to the bracketing that geologists can do with rocks and fossils.
This should be a very brief discussion to help students understand the concept of bracketing by reminding them of the earlier discussion of the family tree. Recall that Question 5 of the earlier discussion showed that the photos provide fairly precise (within a year or two) absolute ages for great-great-grandmother 1 (born around 1917) and grandmother 1 (born around 1957). This allowed us to conclude that great-grandmother 1 must have been born between 1917 and 1957, a range that we could further constrain with assumptions about age of motherhood (e.g., if we assume the mothers are all over age 18, we can narrow the range to 1935 to 1945). For this new discussion, the goal is for students to understand that this thinking process gave us an “age bracket” similar to the brackets that geologists use to constrain the ages of rocks and fossils.
Figure 5.1.3–9 illustrates one common way in which this bracketing can be done. Notice the following key points as you study the figure:
- In this particular case, fossil-containing sedimentary layers are “bracketed by” layers of solidified volcanic ash, which must have been made by volcanic eruptions.
- Volcanic rock is igneous, which means we can find its age through radiometric dating.
- Therefore, once we know the ages of the volcanic layers just above and below a sedimentary layer, we know the age range (“bracket”) of the sedimentary layer and any fossils it contains.
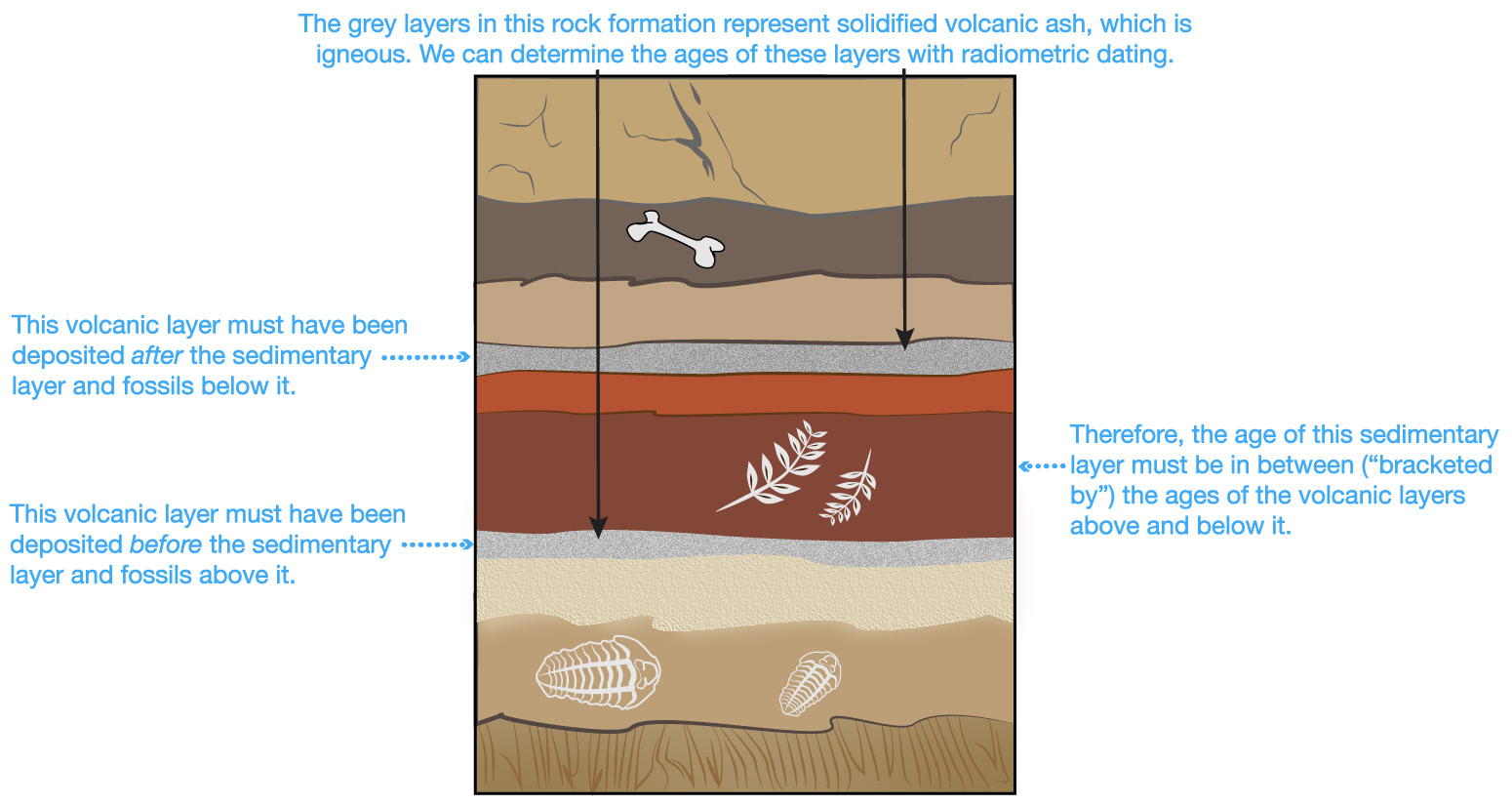
To be sure you understand the idea of bracketing, discuss the following questions with a classmate. Then click to open the answers to see if they agree with what you came up with.
- In figure 5.1.3–9, suppose that radiometric dating tells you that the upper layer of solidified volcanic ash is 467 million years old, and the lower layer is 482 million years old. What can you conclude about the age of the fossils in the sedimentary layer in between those volcanic layers?
- Using the same data, what can you conclude about the age of the fossils in the sedimentary layers that are below both volcanic layers?
- Using the same data, what can you conclude about the age of the fossils in the sedimentary layers that are above both volcanic layers?
Activity
Another Bracketing Example
Bracketing may be possible in different ways for different rocks and fossils. Study the illustration below, then answer the questions that follow.
- Is the sedimentary layer itself older or younger than the rock inclusions?
- Is the sedimentary layer itself older or younger than the igneous dike cutting through it?
- Suppose that you do radiometric dating on one of the rock inclusions and find that its age is 325 million years. Then you do radiometric dating on the igneous dike and find its age to be 295 million years. What can you conclude about the age of the sedimentary layer and the fossils within it?
- Add labels to the figure to summarize what you’ve learned about the highlighted layer.
This activity challenges students to combine the bracketing ideas covered above with some of the relative dating principles they’ve also learned. Notes on the answers:
- (1) Students should recall that inclusions are always older than the surrounding rock. Therefore, the sedimentary layer is younger than its inclusions.
- (2) Students should recall that cross-cutting features like dikes are always younger than the rock they cut through. Therefore, the sedimentary layer is older than the dike.
- (3) Students know from the first two questions that the sedimentary layer is older than the dike and younger than the inclusions. Therefore, we can conclude that the layer is between 295 and 325 million years old. This age bracket also applies to the fossils within the layer.
- (4) The figure below shows one way of adding the labeling.
The Age of the Earth
We’ve said numerous times in this book that Earth is about 4½ billion years old. But how do we know? The answer is through radiometric dating, but perhaps in a somewhat surprising way.
Remember that when we find the “age” or a rock through radiometric dating, we are really learning how long it has been since the rock took on its current form and arrangement of crystals. Because of the rock cycle, rocks on Earth have many different ages, depending on when their form last changed.
This brings us to the first key point about determining Earth’s age: Earth as a whole must be older than its oldest rocks. How old are these rocks? Radiometric dating shows that the oldest intact Earth rocks are about 4 billion years old (Figure 5.1.3–10a/b). However, some rocks contain mineral grains that have been dated to be even older: up to about 4.4 billion years old (Figure 5.1.3–10c). Since the mineral grains must also have crystallized after Earth formed, their ages allow us to conclude that Earth is at least 4.4 billion years old.
Figure 5.1.3–10 – These photos show some of the oldest rocks and mineral grains ever found on Earth.
Of course, the fact that Earth is at least 4.4 billion years old still does not tell us exactly how much older Earth might be. For that, scientists must look beyond the rocks of Earth itself. One way to do this is to study rocks brought back from the Moon by the Apollo astronauts (Figure 5.1.3–11). The oldest Moon rocks found by the astronauts are about 4.44 billion years old. But as with the oldest Earth rocks, this only tells us that the Moon (and Earth) is at least that old.
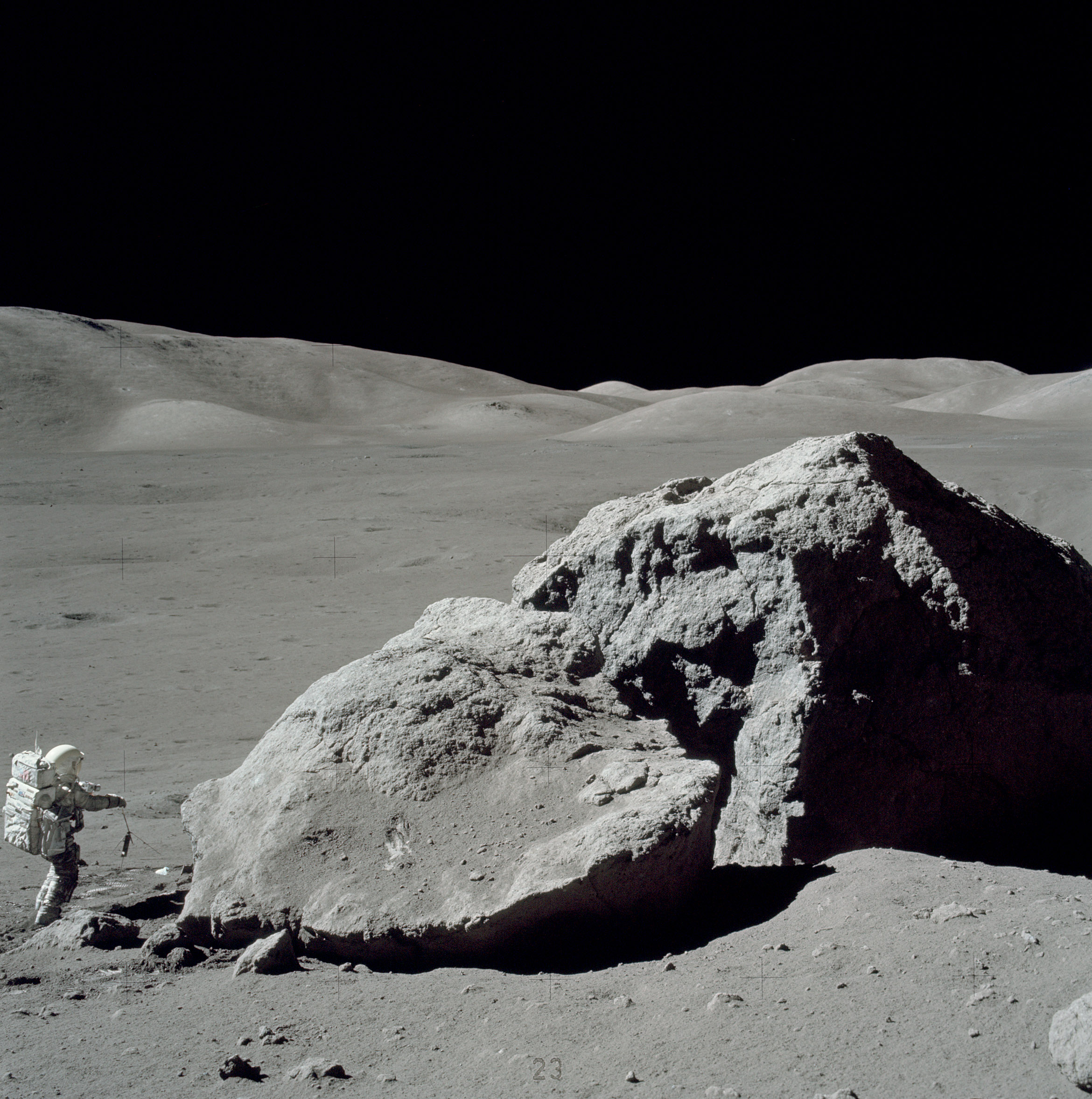
To go all the way back to the beginning of Earth and the solar system, we must find rocks that are unchanged from the very earliest times. If you think back to what you’ve learned about how the planets formed, you’ll realize that we do indeed have such rocks: they are meteorites that have fallen to Earth from space.
Many meteorites are rocks that have not changed since they first solidified in the disk in which the planets formed (Figure 5.1.3–12). Radiometric dating shows that these meteorites formed about 4.56 billion years ago, so this time must mark the beginning of planet formation. Because the planets took a few tens of millions of years to fully form — and 10 million years is 0.01 billion years — we conclude that Earth and the other planets must have formed by about 4.5 billion years ago.
Key Concepts: The Age of the Earth
The Earth as a whole must be older than its oldest rocks and minerals; the oldest intact rocks are about 4 billion years old and some mineral grains are as much as 4.4 billion years old. To get a more precise age for when Earth formed, scientists look to meteorites, many of which represent material that has not changed since the solar system first began to form. Radiometric dating of meteorites leads us to the conclusion that Earth formed about 4.5 billion years ago.
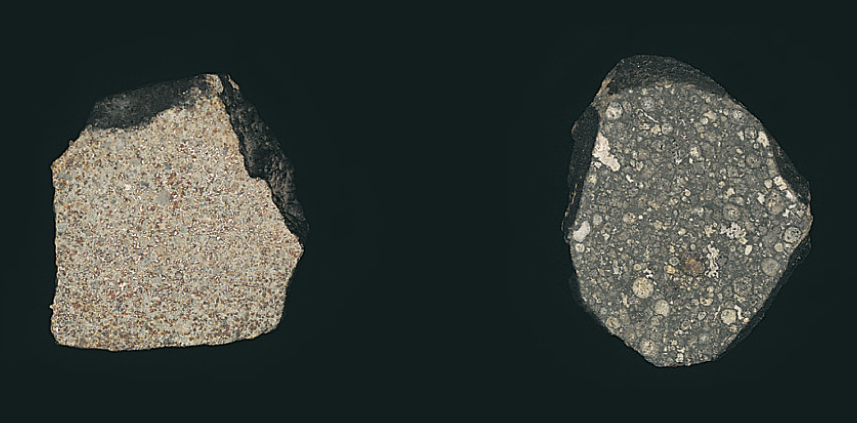
Journal Entry
Rock Photo Journal Part 2
Look back at your journal entry from the beginning of this chapter, in which you took photos of and wrote briefly about at least 3 interesting rock formations near your home or school. Look closely at each one (possibly visiting it again) and write at least one new paragraph in which you describe anything new that you now see or understand about the formation, based on what you’ve learned in this section.
This journal entry builds on the earlier one. The hope is that, having studied rock formation and dating in this section, students will be able to add a little more detail to what they wrote before. Notes:
- Unless the formation is famous enough to have some information about it readily available, students are still unlikely to be able to know much more about it. However, they should still be able to write about things they notice visually now that they might have missed before, such as whether it has any visible inclusions or dikes. If they are having difficulty, encourage them at least to add more detail about color, texture, or other features that they didn’t write about previously.
- If there is available information on the formation, even if students read about it previously, they should be able to interpret it better now. So in this case, encourage students to write about their enhanced understanding.
Optional Video
Discovering the Age of the Earth
If you have time and access to the series, watch the episode “The Clean Room” of the Neil deGrasse Tyson series “Cosmos: A Spacetime Odyssey” (available on various streaming services). After watching the video, hold a brief class discussion about how Clair Patterson’s work in learning the age of the Earth led him to recognize the dangers of leaded gasoline, ultimately leading to the mandate for lead-free gas.
If you have the time, we highly recommend this video because it will not only help students see how we learned the age of the Earth but also covers the socially relevant topic of how the same scientist recognized the danger of leaded gasoline. It should spur very interesting discussion.