To meet our goal of understanding the characteristics that make Earth unique among the worlds of our solar system , we need to study these other worlds in a little more depth. Before we begin, you might wish to quickly review what we’ve already discussed about them:
- Section 1.1.3 describing the basic types of worlds in our solar system.
- Section 1.2.3 describing the scale or our solar system, including the virtual tour of our solar system that you wrote about in a journal entry in that section.
- Section 3.4.3, especially Figure 3.32 and the discussion of why larger bodies are round but smaller are not.
Slide Show 4.47 summarizes some of the key points you should recall from your review.
Slide Show 4.47 – A quick review of what you already know about the diverse worlds of our solar system.
After you have completed your review and studied Slide Show 4.47, you should be ready to begin looking in a little more depth at comparisons between Earth to other worlds. This type of comparison is sometimes called comparative planetology , where “planetology” is used as a generic term not just for planets but for any type of world in space, including moons, asteroids, and comets.
Connections—Etymology
The Many Meanings of “World”
The English word “world” actually has a complex history. If you look back to old English, “world” translates to something like “the affairs of humanity.” However, it is also linked (in a somewhat roundabout way) to the Latin mundus (which is why we translate “the world” in English to el mundo in Spanish) and the Greek kosmos, which means “order” (in the sense of being the opposite of chaos). Of course, kosmos is also the origin of our English word cosmos, which we now take to be another word for the universe.
These many roots and meanings explain why the word “world” is still used in many different ways. Here are just a few examples:
- We sometimes take “world” to refer specifically to Earth; for example, “around the world” means around the Earth.
- We also commonly take it to mean some subset of human experience; for example, the “art world” refers to human-created art, not to the rest of our planet.
- In other cases, we take “world” to include not just Earth but also the rest of the universe; for example, when we talk about your “world view,” we generally mean the way you view your place in the universe.
So what do we mean by “world” in the context of Earth and space science? We gave the following definition back in Chapter 1:
Notice that this definition is essentially a generalization of the first idea above (Earth as the “world”) to include other objects besides our own planet. We include the words “that you could in principle visit” so that we don’t count very small objects (for example, celestial objects the size of rocks or boulders) as “worlds,” since they don’t have enough gravity to allow you to stand on them. Similarly, but on the other end of the size spectrum, we generally don’t think of the Sun or other stars as “worlds,” because their hot, seething surfaces mean they are not places that we could realistically visit.
To sum up, when you hear the word “world” in Earth or space science, you should think of it representing either a planet or any moon, asteroid, or comet that is large enough and has enough gravity so that you could realistically stand on it.
Comparative Compositions
Let’s start by comparing worlds according to composition. If you think about what you learned from your virtual solar system tour, you’ll recognize that worlds fall into three basic categories by composition:
- rocky worlds , which are made mostly from rock and metal.
- hydrogen-rich worlds (sometimes called “gas giants”), which contain a lot of hydrogen, some in the form of molecules of water (H2O), methane (CH4), or ammonia (NH3).
- ice-rich worlds, which contain substantial amounts of ice mixed with rock.
The many worlds of our solar system are not just a random assortment of these three types of composition. Instead, there are clear patterns in which a world’s composition generally depends on its size and distance from the Sun. The following activity will help you understand these patterns.
Claim-Evidence-Reasoning Activity
Size, Distance, and Composition
Work individually or in small groups to do the following.
- On a piece of paper, draw a set of three bins, with one each labeled for “rocky,” “hydrogen-rich,” and “ice rich.”
- Sort the following objects (or types of object) into the correct bins:
Mercury, Venus, Earth, Mars, Jupiter, Saturn, Uranus, Neptune, Pluto, asteroids, comets
You can do this simply by writing the item name in the correct bin on your paper. After you have done this sorting, compare your answers with those of other students. If there is any disagreement, discuss until you come to agreement on the correct sorting. - In a Chapter 1 activity, you created a graph of planetary size versus distance, which should have looked like the following, except we’ve now extended the x-axis to greater distances:
Redraw or print out this graph, then add the following:- a dot for Pluto: diameter = 2,400 km, distance = 5,900 million km
- a shaded area that represents approximately where most asteroids would go on this graph
- a shaded area that represents approximately where most comets would go on this graph
- We have not yet discussed moons in much detail. Below are sizes and compositions for a few major moons in the solar system. Use these data to add these moons into your sorting bins (from step 2 above) and to your graph (from step 3 above). Hint: Remember that a moon is located at essentially the same distance from the Sun as the planet it orbits.
Earth’s Moon: diameter = 3,500 km, composition = rocky
Jupiter’s moon Ganymede: diameter = 5,300 km, composition = ice-rich
Saturn’s moon Titan: diameter = 5,200 km, composition = ice-rich
Uranus’s moon Titania: diameter = 1,600 km, composition = ice-rich
Neptune’s moon Triton: diameter = 2,700 km, composition = ice-rich - Based on what you have found (and further review of the solar system tour as needed), use evidence and reasoning to support each of the following claims:
Claim 1: Only very large worlds can be hydrogen-rich, while small worlds can be either rocky or ice-rich.
Claim 2: Rocky worlds are found only relatively close to the Sun.
Claim 3: The composition of worlds found farther from the Sun depends primarily on their size: very large worlds are hydrogen-rich while smaller worlds are ice-rich.
This CER activity is designed to help students discover for themselves the key ways in which size, distance, and composition are related for solar system objects. The claims at the end contain the key facts that students should discover. This activity can be done individually, but if your students find it challenging it may be helpful for them to work in small groups. Notes on the individual steps:
- Steps (1) and (2) should be very easy, since the answers can be found simply by paging through the solar system tour. The correct answers are:
- Rocky: Mercury, Venus, Earth, Mars, asteroids
- Hydrogen-rich: Jupiter, Saturn, Uranus, Neptune
- Ice-rich: Pluto, comets
- For Step 3, it should be relatively easy for students to plot the point for Pluto with the given data. Shading the areas for comets and asteroids may be more challenging. The answer is shown further below; the key is for students to recognize that:
- Both shaded areas should be vertically short and very close to the x-axis, because asteroids and comets are all smaller than Mercury in size.
- The asteroid area should span much of the distance range between Mars and Jupiter, since that is where the asteroid belt is located.
- The comet area should be wider, starting near Neptune and extending outward. In fact, comets extend very far outward, so in principle this area would extend far beyond the edge of this graph. However, you should accept any answer that shows it spanning a wide range beyond Neptune. Note that it is fine for their Pluto dot to be inside this shaded area, since Pluto’s ice-rich composition means it is essentially an unusually large comet.
- Step 4 should be relatively straight-forward since we provide the needed data. Its purpose is to help students see how moons fit into the scheme of sizes, distances, and composition. Note that we have not previously told them much about moons, though there has been some discussion of Earth’s Moon, and the solar system tour and Slide Show 1.2 (in Section 1.1.3) have introduced students to a few other major moons. Fyi: The moons for which we provide data represent the largest moons of their planets; we chose one from each of the four giant planets so that they’ll be able to place them easily on the graph.
- For the bins: Earth’s Moon should go in the rocky bin, while Ganymede, Titan, Titania, and Triton go in the ice-rich bin.
- For the graph: the moon dots should go directly below the planet dots in each case, since moons are at the same distance as their planets, but much closer to the x-axis because they are much smaller in size.
- The completed graph should look approximately like this:
- Step (5): These claims are the key “discoveries” that students should have made through this activity, so they should be able to cite their work for the evidence and reasoning. In particular:
- Claim 1: Students should be able to support this claim by noting that the only four hydrogen rich objects (as identified in their sorting bins) are also the only four very large worlds (as identified on the graph), while the smaller worlds have the mix of the rocky inner planets along with our Moon and asteroids, and the ice-rich outer solar system moons, Pluto, and comets.
- Claim 2: Again, by comparing the bins and the graph, they should be able to demonstrate that all the rocky worlds are found relatively close to the Sun (more technically, from the asteroid belt inward).
- Claim 3: Here, they should recognize that everything beyond the asteroid belt is either ice-rich or hydrogen-rich, with size being the determining factor since ice-rich moons orbit hydrogen-rich planets.
We can summarize the patterns you’ve found in the activity as follows:
- Rocky worlds are all close to the Sun.
- Ice-rich worlds are all far from the Sun.
- The only hydrogen-rich worlds are the four largest planets (Jupiter, Saturn, Uranus, Neptune), and they are all far from the Sun.
Slide show 4.48 visually summarizes how composition depends on size and distance from the Sun.
Slide Show 4.48 – These slides summarize how a world’s composition depends on its distance from the Sun and its size.
Composition, Oceans, and Life
Our primary goal in this section is to understand why Earth is the only world in our solar system that is home to diverse and abundant life . Part of the reason is clearly due to the fact that Earth is the only planet with surface oceans of liquid water. Liquid water is important to all life on Earth, and the oceans are home to a great deal of this life.
Earth’s rocky composition appears to be an important factor in making it possible for our planet to have its oceans. The four hydrogen-rich worlds (Jupiter, Saturn, Uranus, Neptune) could not have surface oceans because they lack solid surfaces on which oceans could rest. The ice-rich worlds are too cold to have liquid water oceans on their surfaces. (However, some of them probably have oceans hidden beneath their icy surfaces.)
Claim-Evidence-Reasoning Activity
Geospheres and Hydrospheres
Based on what you have learned so far, use evidence and reasoning to support or refute each of the following claims.
Claim 1: The large, hydrogen-rich planets lack geospheres.
Claim 2: Hydrospheres that include surface oceans of liquid water could in principle be found on any rocky or ice-rich world.
This CER activity should be fairly straightforward since it is mostly answered in the above paragraph, but it will still serve at least three purposes: (1) it reviews student understanding of the terms geosphere and hydrosphere; (2) it gets them to consider the question of whether or which other worlds have similar “spheres”; (3) it forces them to summarize for themselves the reasons why we think that Earth’s rocky nature is an important part of its uniqueness. Note that to some extent, it is legitimate for students to argue either way on these claims, so focus your evaluations on their evidence and reasoning more than if they chose the “right” answer.
- For the first claim: As defined earlier, the geosphere represents the solid surface of Earth and everything interior. In that sense, students can cite the lack of solid surfaces on the four large planets (Jupiter, Saturn, Uranus, Neptune) to support the claim. Note, however, that students who remember that these planets are actually liquid throughout much of their interiors (see this box) might suggest that we should refer to the parts below their gaseous atmospheres as their geospheres; this is a legitimate suggestion, even if it is not the way the term geosphere is usually used. (The liquid layers of these planets consist mainly of liquid hydrogen, not water, so these would not count as a hydrosphere, since the “hydro” in hydrosphere refers to water, not hydrogen).
- For the second claim: Generally speaking, students should reject this claim (but see the caveats below for those who might not) because:
- The ice-rich worlds have icy surfaces because they are far from the Sun, which means they are too far away to have liquid water oceans on their surfaces. Caveats: (1) Some of these worlds are thought to have subsurface oceans (e.g., Europa, Enceladus); although this is not what the claim was talking about, it is legitimate to think of these as subsurface hydrospheres. (2) As discussed in the Wow Factor box below, other solar systems may have planets (the so-called “water worlds”) that have compositions that essentially match those of ice-rich worlds but that are warm enough for the “ice” to be in liquid form.
- For rocky worlds, students already know that none of the other rocky worlds of our solar system (besides Earth) have oceans. They therefore know that hydrospheres are not a general feature of rocky worlds. Caveat: Whether a rocky world could “in principle” have a hydrosphere depends on how those words are interpreted. As we’ll discuss below, it seems likely that a rocky world of sufficient size and at the right distance from its star (in the so-called habitable zone) might in fact be expected to have liquid water oceans. So if students take “in principle” to mean “given the world’s actual size and orbit” then the claim is false; however, if they take “in principle” to mean “assuming it has the right size and orbit” then the claim could be true for rocky worlds (but still not for ice-rich worlds).
Wow Factor
Super-Earths, Water Worlds, and hot Jupiters
Understanding WHY Composition Depends on Size and Distance from the Sun
This subsection is optional, as it goes beyond what is generally expected in middle school science (and is not covered in NGSS for middle school). However, it is not that difficult to understand, and it should answer questions that at least some students will naturally wonder about, given what we have discussed about patterns of composition.
You might wonder why a world’s composition depends on its size and distance from the Sun in the way we’ve found. Scientists trace the answer to processes that occurred at the time the solar system was first forming, about 4½ billion years ago.
It’s easiest to understand the explanation if we begin by ignoring the four very large hydrogen-rich planets (Jupiter, Saturn, Uranus, Neptune). In that case, we see a simple pattern with distance from the Sun:
Close to the Sun we find rocky worlds, and farther from the Sun we find ice-rich worlds.
You can probably guess that this must have something to do with temperature, since ice can stay frozen only at low temperatures and we generally expect temperature to be lower at greater distances from the Sun. However it isn’t so much temperatures today that matter, but temperatures at the time the solar system was forming.
Recall from Section 4.1.3 that, according to the modern scientific model of solar system formation, the young Sun was surrounded by a spinning disk of gas and dust. Within that disk, the formation of planets began with small, solid particles combining together to make larger ones (see in Figure 4.21). The largest particles grew to become the planets, while smaller “leftovers” became asteroids and comets.
Figure 4.49 generalizes this model to also show how the small, solid particles would have differed in the inner and outer regions of the solar system:
- Close to the Sun, where it was fairly hot, only rocky material (which includes both rock and metal) could make the solid particles. It was too hot for molecules of water (H2O), methane (CH4), or ammonia (NH3) to freeze into ice; instead, the heat of the Sun kept them gaseous.
Therefore, worlds that formed close to the Sun must have formed from these rocky particles, explaining the rocky composition of the inner planets and of asteroids. - Farther from the Sun, where it was much colder, the solid particles included ice as well as rocky material.
Therefore, worlds that formed far from the Sun formed out of rocky and icy particles, explaining the ice-rich compositions of the moons of outer planets and of comets.
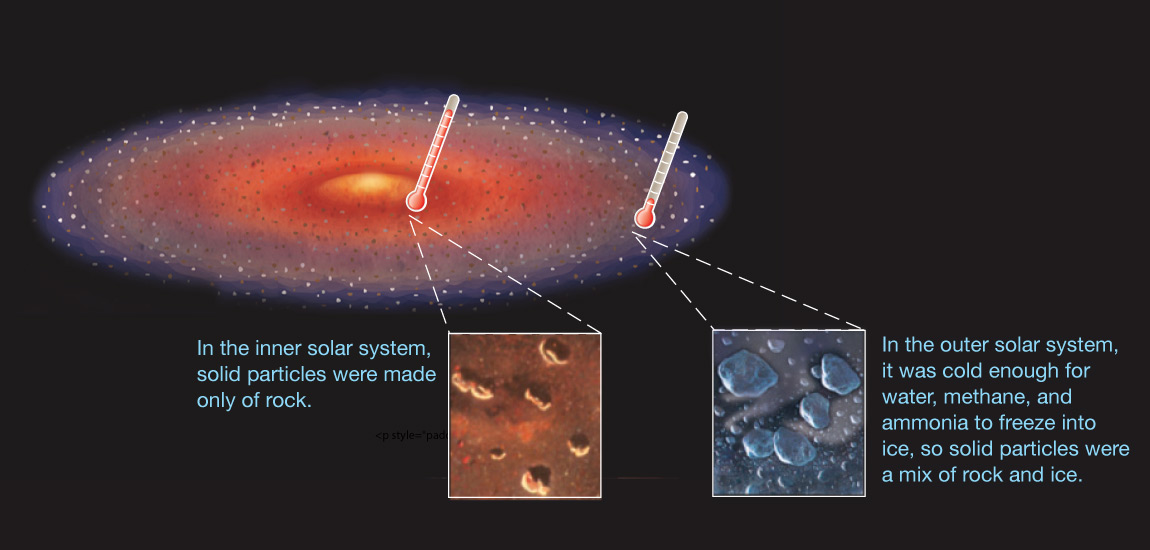
The model shown in Figure 4.49 explains why inner solar system worlds tend to be rocky and why small outer solar system worlds (moons and comets) tend to be ice-rich. But how do we explain the four large, hydrogen-rich planets (Jupiter, Saturn, Uranus, Neptune)?
We can trace the compositions of these planets to their large sizes, but first you need to know an important fact about the disk in which the planets formed: The disk was made mostly of gas. In fact, observations of other stars and gas clouds tell us that gas must have made up about 98% of the mass of the disk; only about 2% took the form of solid particles. Most of this gas (about ¾ of it) was hydrogen, and the remainder (about ¼ of it) was mostly helium. The vast majority of the particles in the disk, including those that grew to become Earth and the other rocky planets, did not have strong enough gravity to pull in and hold onto any of this gas. However, this situation could change if a particle grew large enough.
Figure 4.50 summarizes how scientists add these ideas to the earlier model, explaining the origin of the hydrogen-rich planets as follows:
- The fact that particles in the inner solar system were made only from rock, while particles in the outer solar system were made from both rock and ice, meant there was more solid material in the outer solar system.
- This in turn made it possible for at least some ice-rich particles in the outer solar system to grow to much larger sizes than was possible for the rocky particles of the inner solar system. The fact that there are four large planets in the outer solar system today tells us that there must have been four ice-rich “particles” that grew quite large .
- These four very large ice-rich particles had such strong gravity that they began to pull in hydrogen (and helium) gas from the surrounding disk, and the more gas they pulled in, the stronger their gravity became, allowing them to pull in even more.
- Ultimately, these four particles pulled in so much hydrogen that it changed their overall compositions from ice-rich to hydrogen-rich.
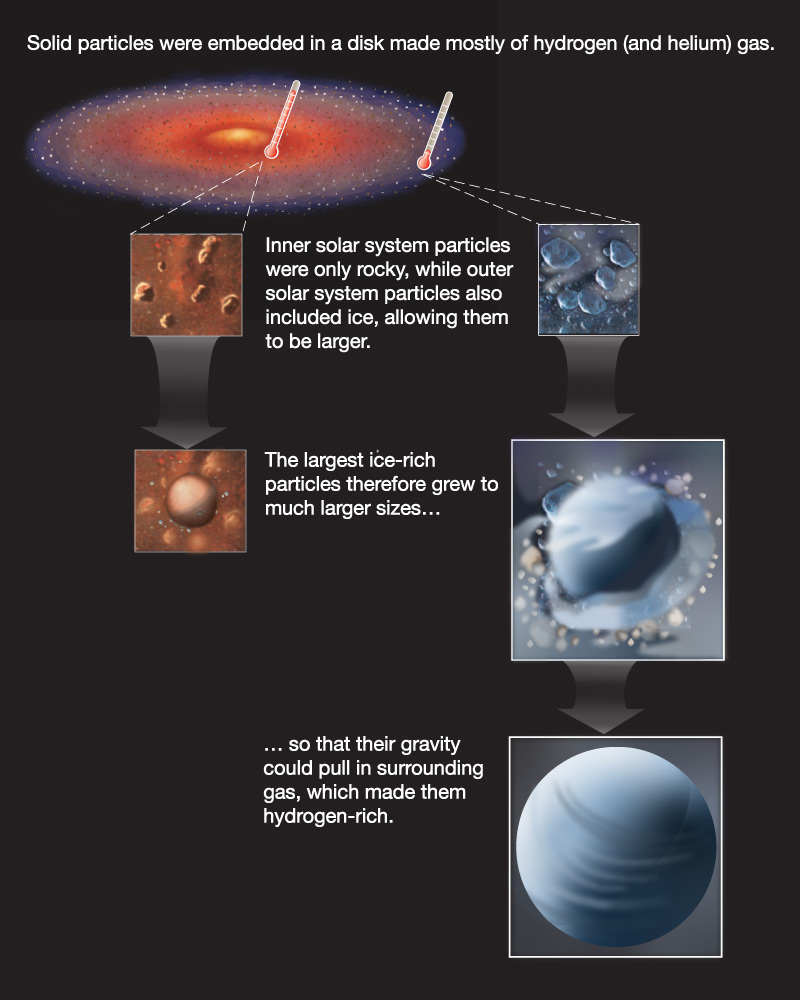
Discussion
Differences among the hydrogen-rich planets
Although all four of the outer planets are hydrogen-rich, they can be separated into two subgroups: Jupiter and Saturn make one group, while Uranus and Neptune make the other. The two major differences between these subgroups are:
- Mass: Jupiter and Saturn are much more massive than Uranus and Neptune.
- Detailed composition: Jupiter and Saturn are made mostly of “pure” hydrogen and helium, while Uranus and Neptune have a much more substantial proportion of the hydrogen-containing molecules that make ices when frozen (such as water, methane, and ammonia).
Working in small groups, consider what you’ve learned in this section and try to come up with a hypothesis to explain these general differences between Jupiter/Saturn and Uranus/Neptune. Then have a representative from each group present the hypothesis to the class, and work as a class to try to come up with a consensus hypothesis. Your teacher will then tell you whether you’ve come up with the same explanation that most scientists think to be likely.
This is an optional — and challenging — discussion topic. However, if you feel your students are up to it, it will provide an excellent opportunity for students to form and evaluate their own hypotheses, to try to come up with a group consensus, and to consider one of the many mysteries that remain in our quest to understand planetary formation. In terms of talking with students about the “correct” answer:
- The generally accepted hypothesis begins with a fact noted in the information bubble that appears in the first bullet point above the Discussion: Before they began pulling in gas, models suggest that all four of the ice-rich particles that became the outer planets had masses about 10 times the mass of Earth.
- This means that all the rest of the masses of these planets came from the hydrogen and helium gas that they pulled in.
- Which in turn makes it easy to see how the masses correlate with the compositions:
- Jupiter and Saturn have masses far larger than 10 times that of Earth (318 and 95 times, respectively). This means they pulled in so much hydrogen and helium gas that the material of their original ice-rich particles ended up as only a small fraction of their total masses.
- Uranus and Neptune have masses only 14 and 17 times that of Earth, respectively. This means that the water, methane, and ammonia that made up much of the masses of their original ice-rich particles still represents more than half of their total masses, which is why these hydrogen compounds represent a large portion of their overall compositions. (Note that, as discussed in the last paragraph of this box, the temperatures and pressures in the interiors of these worlds mean that these molecules are no longer in the form of ice.)
- Note, however, that the above “answer” still leaves a major question: If all four planets started from ice-rich “seeds” of the same size, why did they end up with such large differences in how much gas they pulled in? The answer to this question is not yet known. It probably had something to do with the fact that the density of the gas should have declined with distance from the Sun. For example, this would explain why the innermost of these worlds, Jupiter, grew the largest. But it doesn’t explain why there would be such a large drop-off from Saturn to Uranus, nor does it explain why Neptune is more massive than Uranus. Clearly, we still have much more to learn about the details of how the planets formed.
Comparative Atmospheres (Rocky Worlds)
Because we know that Earth’s oceans are important to its extensive biosphere, and because only rocky worlds seem likely to have surface oceans, we will now focus our attention on the rocky worlds of our solar system. We’ll start with their atmospheres. As you will see, it wouldn’t be possible to have oceans without a fairly substantial atmosphere like that of Earth.
Atmospheric Pressure
The most basic question we might ask about an atmosphere is: How much atmosphere does a world have? Scientists usually answer this question in terms of the pressure with which the atmosphere pushes on the surface, which we call the atmospheric pressure . (We’ll discuss how this pressure is measured in Chapter 6.)
The following table summarizes the distances, diameters, and atmospheric pressures of the rocky worlds (going outward from the Sun). All the values are shown relative to Earth. For example, the “90” for Venus’s atmospheric pressure means that it is 90 times the atmospheric pressure on Earth.
World(s) | Distance from Sun (Earth=1) | Diameter (Earth=1) | Atmospheric Pressure (Earth=1) |
---|---|---|---|
Mercury | 0.39 | 0.38 | 0 |
Venus | 0.72 | 0.95 | 90 |
Earth | 1 | 1 | 1 |
Moon | 1 | 0.27 | 0 |
Mars | 1.52 | 0.53 | 0.007 |
Asteroids (in asteroid belt) | 2 to 3 | <0.1 | 0 |
Quick Quiz – Interpreting the Table
Answer the following questions to make sure you understand the numbers in the table.
Note: As always, after you finish the quiz, be sure you read the feedback that explains the correct answers, to make sure you understand the reasons for these answers.
Now that you understand how to interpret the table, take a few minutes to study it in search of any features or patterns. The following questions should help you in this process.
This set of questions is designed to help students recognize that whether or not a world has an atmosphere depends primarily on the world’s size: As we discuss after the questions, only relatively large worlds have strong enough gravity to hold on to the gas that makes up an atmosphere. We ask students to work through these questions to recognize this fact because, as addressed especially in the last two questions, it is not immediately obvious from the table. The issue is that if you look only at the worlds with atmospheres, there is no relationship to size; for example, Venus and Earth are nearly the same size but have very different atmospheric pressure. It’s only when you look for an abrupt change from “no atmosphere” to “atmosphere” that you recognize that having an atmosphere is indeed dependent on having some minimum size.
Discuss the following questions with a classmate, then click to open the answers to see if they agree with what you came up with.
- The table shows that Mercury, the Moon, and asteroids all have zero atmospheric pressure. What is this telling us?
- Which of the rocky worlds in our solar system have atmospheres? List them in order from lowest to highest atmospheric pressure.
The table shows that only Venus, Earth, and Mars have nonzero atmospheric pressure, which means these are the only three worlds with atmospheres. Venus has the highest atmospheric pressure (90 times Earth), and Mars has the lowest (0.007 times Earth), so the order from lowest to highest atmospheric pressure is: Mars, Earth, Venus.
- Does the table show any clear relationship between atmospheric pressure and distance from the Sun?
No. There are at least two easy ways to recognize this lack of relationship between atmospheric pressure and distance:
- Earth and the Moon are both at the same distance from the Sun, but Earth has an atmosphere and the Moon doesn’t. This alone rules out a simple relationship between atmospheric pressure and distance.
- The atmospheric pressure numbers neither increase nor decrease in the same order as distance. For example, the pressure (relative to Earth) goes up from zero at Mercury to 90 at Venus, but then down to 1 at Earth (and 0 at the Moon).
- Looking for a relationship between atmospheric pressure and size is a bit more subtle, so we’ll consider two questions. First, do you see a relationship between pressure and size if you look only at the three worlds with atmospheres (Venus, Earth, and Mars)? Explain.
- Next, remember that there are a lot of asteroids; in fact, thousands that are large enough to think of as “worlds.” Therefore, even though there are only 6 rows in the table, the data actually represent thousands of worlds. With this in mind, fill in the blank:
Out of the thousands of rocky worlds in our solar system, the only three that have an atmosphere are the three _____ of these worlds.
The fact that only the three largest of the thousands of rocky worlds have an atmosphere is clearly telling us that size must play an important role in whether or not a world has an atmosphere. At the same time, the fact that Venus and Earth are nearly the same size but have very different atmospheric pressures tells us that other factors must also play a role. We’ll investigate some of those “other factors” later (especially in Chapter 7). But for now, notice the pattern that we see if we divide the rocky worlds into three categories by size (Figure 4.51):
- Small rocky worlds (Mercury, the Moon, and asteroids) have no atmospheres.
- Mars, which scientists consider to be the one and only “medium-size” rocky world in our solar system, has an atmosphere, but not very much of one, since its atmospheric pressure is only 0.007 times that of Earth.
- The two “large” rocky worlds (Venus and Earth) both have substantial atmospheres.
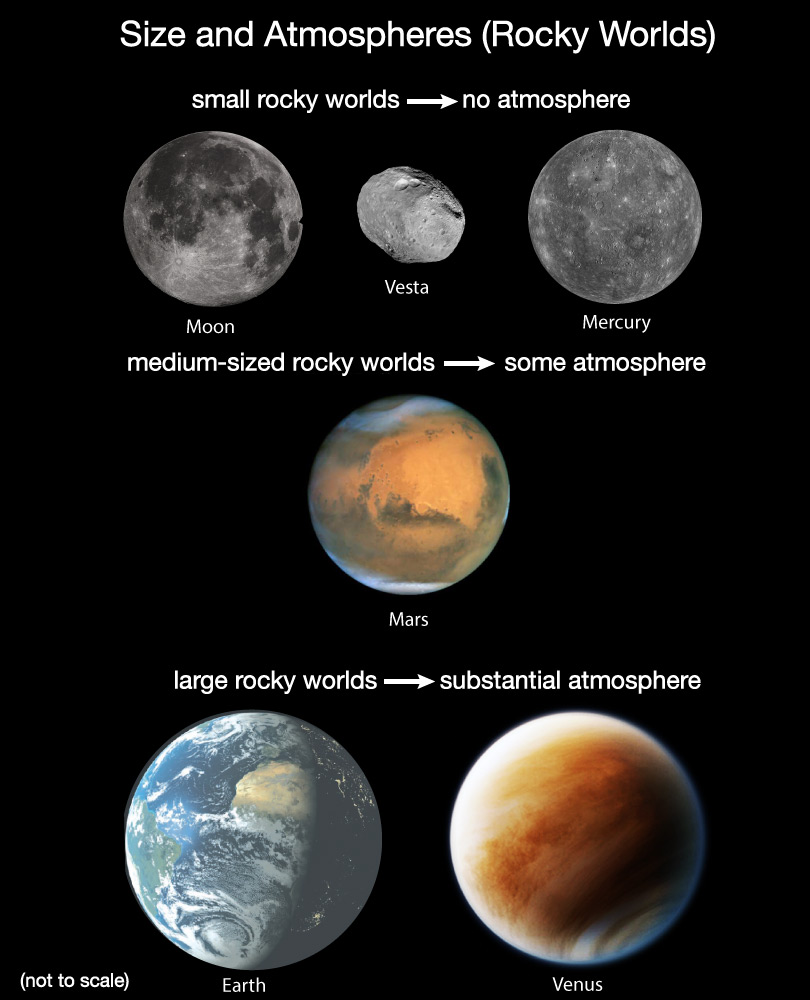
The Linkage Between Size and Atmospheric Pressure
Why do we see this pattern linking size and atmospheric pressure for the rocky worlds? There are two major reasons. The first is one that you should already understand based on what you have learned about size and gravity.
Discussion
Size, Gravity, and Atmospheres
How would you expect the strength of gravity to differ on rocky worlds of different sizes? Explain why, and then discuss how this can help explain the linkage between size and atmospheres for the rocky worlds.
This discussion should be fairly simple. The key evidence that students should recall is that, generally speaking, larger worlds have stronger gravity. This explains the pattern as follows:
- The small worlds, which all have weak gravity, have no atmosphere.
- The large worlds (Venus and Earth) have much stronger gravity and both have substantial atmospheres.
- Mars fits this pattern as the intermediate case: Its intermediate size apparently gives it enough gravity to hold some atmosphere, but not enough gravity to hold an atmosphere as substantial as those of Venus and Earth.
For your own background, and in case student questions arise, there are a few subtleties you should be aware of:
- Technically speaking, the strength of gravity depends on both the size and mass of a planet (in accord with Newton’s universal law of gravitation). However, we are generally able to ignore the mass and focus on size because rocky worlds all have similar densities. Still, if you get into the data, there’s an exception even here: Mercury’s large core gives it a relatively high average density, and as a result, Mercury and Mars actually have nearly identical surface gravities despite Mercury’s smaller size.
- You (and some of your students) might wonder if there is some “minimum” size that makes it possible to have an atmosphere. The answer is that we can’t define that clearly, because of other factors that also play a role. More specifically:
- Whether a world can hold an atmosphere essentially depends on whether the gas molecules in its atmosphere are moving fast enough for some of them to reach the planet’s escape velocity.
- The escape velocity depends only on the world’s gravity, which is why the strength of gravity is so important to whether or not a world has an atmosphere.
- However, the speeds of gas particles depend on both their masses (lighter molecules, like hydrogen, travel faster than heavier molecules, such as oxygen or nitrogen) and on the temperature (higher temperatures mean higher speeds).
- Therefore, whether a world of a particular size can hold onto an atmosphere depends not only on its mass but also on the composition of any atmospheric gas and on how far the world is from the Sun.
- The above ideas come into play for Saturn’s moon Titan (discussed in an upcoming Wow Factor box): Although Titan is larger in diameter than Mercury, its surface gravity is significantly weaker because it has much lower density as an ice-rich world. Nevertheless, Titan is able to hold on to its atmosphere because of its very low temperature (a consequence of its great distance from the Sun), which means its gas molecules move too slowly to reach escape velocity.
- Another subtlety arises from the fact that the solar wind (particles that the Sun expels into space) can essentially knock gas molecules out of a planet’s atmosphere. In fact, as we’ll briefly discuss in Section 4.3.2, Mars lost much of its atmospheric gas in this way, because it lacks a global magnetic field to protect the atmosphere from the solar wind. However, this is still ultimately a consequence of Mars’s relatively small size as follows:
- A strong global magnetic field like Earth’s is generated by the convection of molten metal in the core (in Earth’s case, in the molten outer core).
- This means that having a strong global magnetic field requires substantial internal heat; otherwise, the necessary convection would stop.
- Mars had enough internal heat and therefore had a magnetic field early in its history. However, its relatively small size means that Mars’s interior cooled much more rapidly than Earth’s (just as a small hot potato cools more rapidly than a large one). Evidence indicates that, by about 3 billion years ago (or earlier), Mars had lost enough heat that the necessary core convection ceased, so that Mars lost its magnetic field.
- To sum up: Mars’s current lack of a protective magnetic field is attributable to its size being much smaller than Earth, just like its weaker strength of gravity.
As you’ve hopefully realized through the above discussion, the stronger gravity of larger worlds makes it easier for them to hold on to an atmosphere. That is the first major reason for the linkage between size and atmospheres.
The second major reason for the linkage between size and atmospheres has to do with where the rocky worlds got their atmospheric gas in the first place. As we’ve discussed, even the largest rocky worlds (Earth and Venus) were too small to have enough gravity to pull in hydrogen (or helium) gas from the surrounding disk as they formed. Instead, the gas that formed the original atmospheres of Venus, Earth, and Mars is all thought to have been released from their interiors through a process called outgassing , primarily by volcanoes (Figure 4.52). You already know that volcanoes are driven by energy from internal heat, and as we’ll discuss shortly, larger worlds have more internal heat than smaller ones. For this reason, the amount of outgassing was also linked to size, with more outgassing on larger worlds.
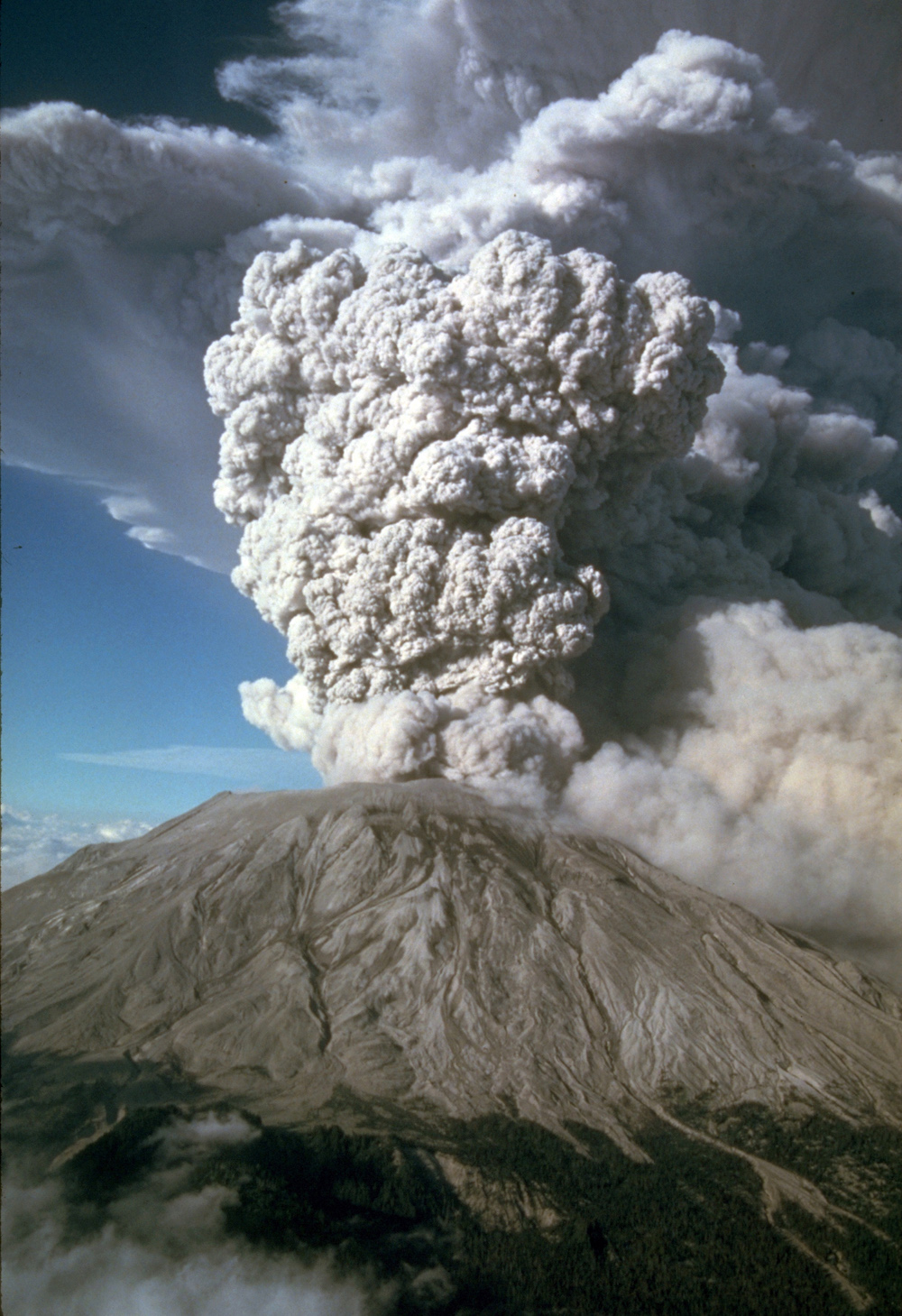
Atmospheric Pressure and Surface Water
The amount of atmosphere on a world has a direct bearing on whether the world can have surface liquid water, such as lakes, rivers, or oceans. More specifically, if a world does not have enough atmosphere, then any liquid water would either freeze or boil away quite quickly.
This is exactly what happens on Mars today: Imagine living in an enclosed colony on Mars. If you poured yourself a cup of water, then put on a spacesuit and walked outside, all the water in your cup would quickly either freeze or evaporate (Figure 4.53a). Moreover, if you then heated the ice that remained frozen in your cup, it would turn directly into water vapor, without first melting into liquid water — just as “dry ice” (frozen carbon dioxide) does on Earth (Figure 4.53b).
Figure 4.53 – Mars has such a thin atmosphere that liquid water cannot exist for more than a few minutes outside on the surface. From this idea, we know that the abundant liquid water on Earth’s surface would not be possible without our planet’s much more substantial atmosphere.
The same general idea applies to all other worlds. For example, even if future astronauts somehow brought lots of water to the Moon, they could not put that water into any kind of outdoor pool, since it would rapidly either freeze or boil away. (They could, however, build a pool inside a pressurized colony.) That is one of the reasons that we don’t see liquid water on the surfaces of any worlds that lack atmospheres.
Claim-Evidence-Reasoning Activity
Size and Atmospheres
Based on what you have learned about Mars, use evidence and reasoning to support or refute the following claim.
Claim: Earth would still have its oceans even if the atmospheric pressure dropped to become as low as that on Mars.
Wow Factor
Titan: A Moon with an Atmosphere
Atmospheric Composition and Temperature
Atmospheres can differ not only in pressure but also in their compositions and in the surface temperatures that they help a planet to maintain through the greenhouse effect (which we’ll study in Chapter 7). The following table repeats the earlier data for Venus, Earth, and Mars, but this time with added columns for surface temperature and atmospheric composition.
World(s) | Distance from Sun (Earth=1) | Diameter Earth=1) | Atmospheric Pressure (Earth=1) | Average Surface Temperature | Dominant Gases |
---|---|---|---|---|---|
Venus | 0.72 | 0.95 | 90 | 470o C | carbon dioxide (96%) |
Earth | 1 | 1 | 1 | 15oC | nitrogen (78%) oxygen (21%) |
Mars | 1.52 | 0.53 | 0.007 | -55oC | carbon dioxide (95%) |
Discuss the following questions with a classmate, then click to open the answers to see if they agree with what you came up with.
- Would it be possible for us to breathe the air on either Venus or Mars?
- Venus and Mars have nearly identical percentages of carbon dioxide in their atmospheres. Does this mean that both planets have the same total amount of carbon dioxide? Why or why not?
It does not, because as you learned above, the best measure of the “amount” of air in an atmosphere is the atmospheric pressure. Therefore, the fact that Venus has far greater atmospheric pressure tells us that it must have far more total gas in its atmosphere, and since both planets have the same percentage of carbon dioxide, Venus must have far more total carbon dioxide.
The differing amounts of carbon dioxide turn out to be very important to understanding the differing temperatures of the three worlds — but that’s a topic we’ll save for Chapter 7, since understanding it will help us understand the issue of global warming.
Discussion
Visiting Venus
People talk a lot about the possibility of sending astronauts to Mars within the next couple decades. Why don’t we hear the same thing about sending people to Venus? Briefly discuss some of the challenges of visiting Venus. Do you have any ideas that might make it possible in the future?
This can be a very brief discussion in which students recognize that Venus’s high temperature and pressure would create an enormous challenge for sending people to the planet. A few notes:
- The temperature poses the most obvious challenge. You’d need very efficient cooling in order to avoid a near-instant death from the heat alone.
- The pressure is also an enormous problem, as it is equivalent to that nearly a kilometer beneath the ocean surface on Earth.
- The thick atmosphere has a density about 10% that of water. That’s much too low for you to float, but it would offer noticeably more resistance to motion than air on Earth. This would be particularly challenging when you wanted to go home, because the air resistance would make it much more difficult to launch a rocket from Venus than from Earth.
- With regard to students coming up with ideas that might make a visit possible: We certainly don’t expect students to come up with viable plans, as no one has been able to do that so far. However, it should make for a fun challenge for them to begin to think about what might be involved. Fyi, while there has been little consideration of sending people to Venus, scientists have been considering ways in which we might get a robotic spacecraft to survive there for an extended period of study (that is, much longer than the Russian landers in the 1970s and 1980s that lasted less than a couple hours). For background information, you can do a search on the Russian space agency’s “Venera-D” mission concept.
Distance from the Sun: The Habitable Zone
Fyi, you and your students may sometimes hear the habitable zone referred to instead as the “Goldilocks zone,” a name that comes from the English fairy tale of Goldilocks and the Three Bears (in which the little girl named Goldilocks finds the baby bear’s porridge, chair, and bed to be “just right”). This can be a cute name to discuss with your students, but be aware that this fairy tale is rarely familiar to students whose first language is not English or who have parents from outside of the United States or Great Britain.
Although atmospheric composition is important to temperature, it should be fairly obvious that distance from the Sun also matters. In particular, if you took a planet with its current atmosphere and moved its orbit closer to the Sun, it would warm up. If you moved its orbit farther from the Sun, it would cool down. This means that there must be some range of distances around the Sun — or any other star — in which it is possible for a world to have temperatures that allow for liquid water on its surface. The region that represents this range of distances is called the habitable zone of the star. In other words, surface oceans are potentially possible within the habitable zone, but not possible outside it.
Figure 4.54 shows current scientific estimates for the habitable zone of our solar system. Note that:
- Earth is clearly in the habitable zone, as it must be since our planet has oceans.
- Venus is in the region where it is too hot for a planet to have surface oceans, no matter what type of atmosphere it has .
- Mars is in a region that is probably within the habitable zone , though scientists are not completely sure. Beyond the habitable zone, it would be too cold for any rocky world to have surface oceans.
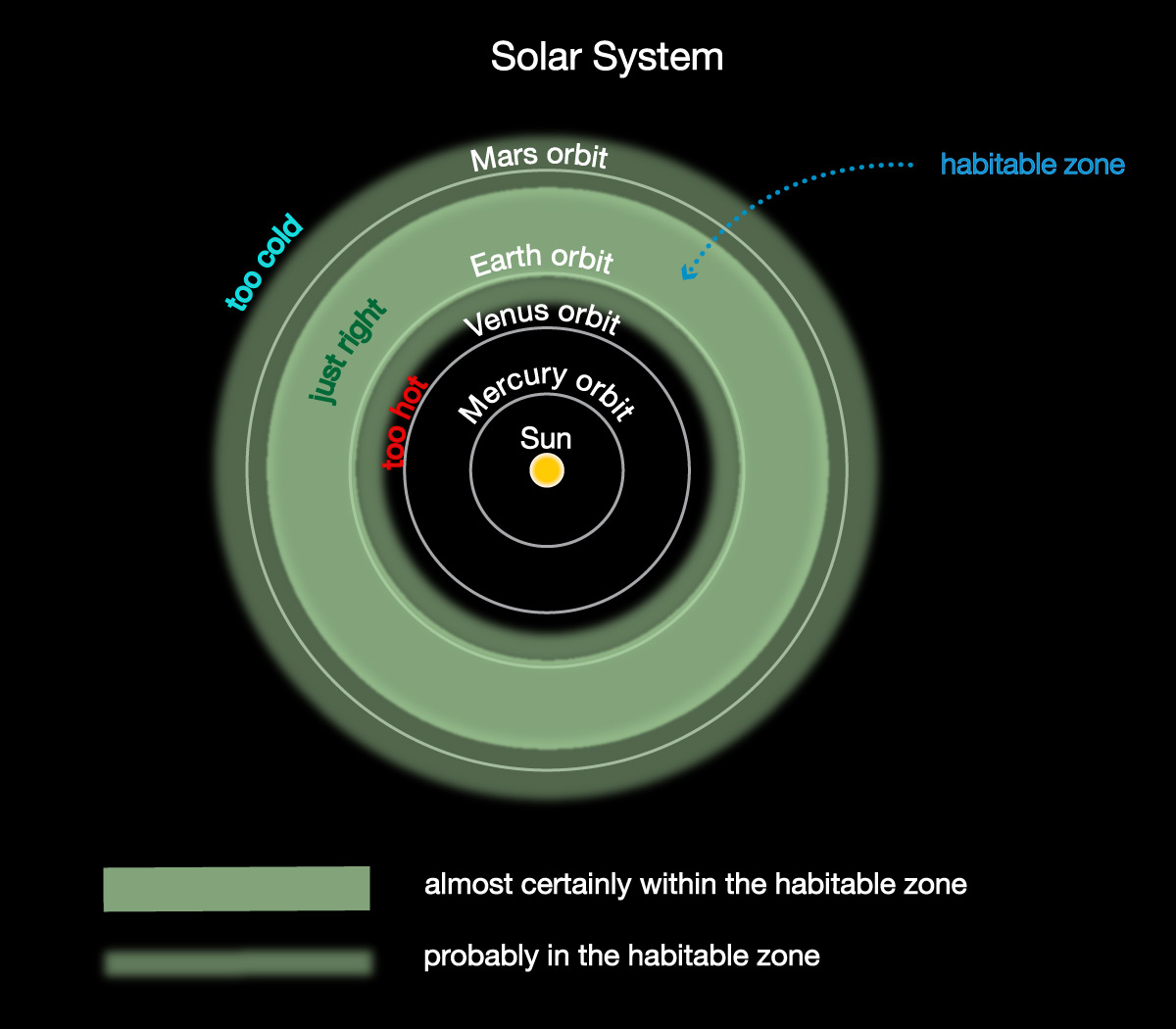
Claim-Evidence-Reasoning Activity
The Habitable Zone and Mars
1. Use the fact that the Moon lacks oceans to support the following claim:
Claim: Being in the habitable zone is necessary for having oceans, but not sufficient for having them.
2. The fact that all rocky worlds have similar compositions means that they differ from one another primarily in just two fundamental properties: their sizes and their distances from the Sun. Which of these two properties of the Moon can explain its lack of oceans? Explain how.
3. Let’s assume that Mars is in the habitable zone (even though Figure 4.54 shows it’s only “probably” in it). Let’s further assume that Mars once had a surface ocean, long ago, as some studies of Mars suggest. (Mars almost certainly had surface liquid water in the past, but there is debate over whether it had an ocean.) Based on what you’ve learned, use evidence and reasoning to explain why the following claim is then reasonable, even if not certain:
Claim: If Mars were as large as Earth, it might still have an ocean today.
This is a challenging activity that, if you do it, will help students understand the next section about what makes Earth a planet with life. A few notes:
- (1) The definition of the habitable zone implies that it is necessary to be in it to have oceans. The Moon is in the habitable zone (since it is at the same distance from the Sun as Earth), so its lack of oceans therefore proves that being in the zone is not sufficient to have oceans. Note: If your students are not familiar with the necessary/sufficient phrasing, then you may need to discuss that before they do this part.
- (2) Students have already learned that the Moon lacks an atmosphere because of its small size, which leaves it with gravity too weak to hold on to atmospheric gases.
- (3) This one will be particularly challenging because of the scientific uncertainties acknowledged in it. The key reasoning required is to notice that the above ideas show that size is a critical factor to having enough atmosphere to have liquid oceans. Earth is obviously large enough. Therefore, if Mars is indeed in the habitable zone, and if it indeed had an ocean in the past, then being as large as Earth would seem to give a strong likelihood that it would still have that ocean today.
Summary of how Atmospheres Depend on Size and Distance
We have found that a world’s size and distance from the Sun both play important roles in the atmospheres of the rocky worlds. Figure 4.55 provides a visual summary, and we can also summarize the ideas in words:
Size determines whether a world is likely to have an atmosphere at all:
- Small rocky worlds have gravity too weak to hold an atmosphere.
- Large rocky worlds (like Venus and Earth) can hold a substantial atmosphere, though there may still be significant differences in atmospheric pressure (as there is with Venus and Earth).
- Medium-size rocky worlds (like Mars) are likely to be in-between cases.
Distance from the Sun determines whether the surface temperature could be in a range that would allow surface oceans to exist:
- A world that is too close to the Sun would be too hot, even if it has an atmosphere.
- A world that is too far from the Sun would be too cold, even if it has an atmosphere.
- A world that is within the habitable zone could in principle have temperatures that allow for surface oceans of liquid water.
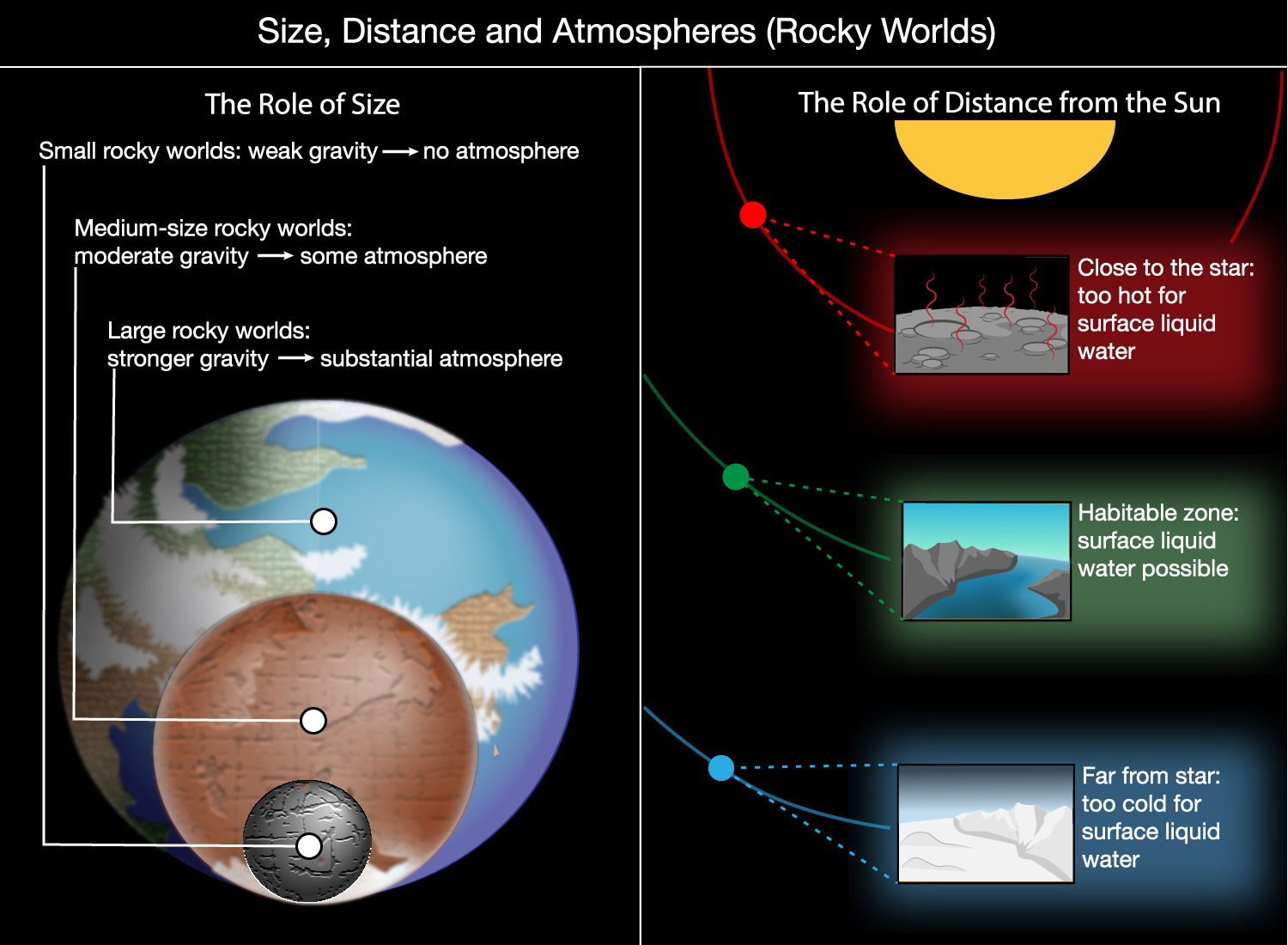
Discussion
Habitable Zones Around Other Stars
Just as there is a habitable zone around our Sun in which temperatures could potentially allow for surface oceans, there are similar habitable zones around other stars. However, not all stars are exactly alike: Some are hotter and brighter than our Sun, others are cooler and dimmer than our Sun. How would you expect the habitable zone of a hotter and brighter star to differ from the habitable zone around our Sun? What would you expect for the habitable zone around a cooler and dimmer star? Explain your reasoning.
The key to this discussion is recognizing that a hotter and brighter star would produce more solar energy and a cooler and dimmer star would produce less. As a result:
- The habitable zone of a hotter, brighter star starts farther from the star and extends over a wider range of distances than the habitable zone of our Sun.
- The habitable zone of a cooler, dimmer star is narrower and closer-in than the habitable zone of our Sun.
The figure below shows this idea conceptually.
Comparative Geospheres (Rocky Worlds)
We turn next to comparing the geospheres of the rocky worlds. As with Earth, any other world’s geosphere includes both its interior and its surface. We’ll consider the interiors first.
Rocky Interiors
Recall that we know Earth has a layered interior (core-mantle-crust) because its average density is so much larger than the density of surface rocks (see Section 4.1.2). Similar differences between average density and surface density are found for the other inner planets and the Moon, which implies that they also have core-mantle-crust structures. Figure 4.56 shows the estimated sizes of these layers in these worlds.
You will also recall that Earth’s internal layering tells us that its interior must once have been
molten, so that the materials could separate by density (the process called differentiation ).
We conclude that the same must also be true for the other rocky planets and the Moon. In other
words, the five worlds shown in Figure 4.56 must all once have been so hot inside that their
interiors melted.
In contrast, small asteroids don’t appear to have any internal layering, implying that if you cut them open, their insides would look similar to their outsides. This tells us that small asteroids were never very hot inside. (This will make sense if you look back the I Was Wondering box that discusses how Earth got hot inside. Asteroids are so small that very little gravitational potential energy was available to be converted into heat.)
Impact Craters and Volcanoes
We now turn our attention to the surfaces of the rocky worlds. Scientists can learn a lot by studying details of surface features, but the first two things you might look for are:
- Does the surface have a lot of impact craters , like those we see on the Moon (see Figure 4.22)?
- Does the surface have active volcanoes (meaning volcanoes that have either erupted recently or are likely to erupt again in the future)?
Slide Show 4.57 shows representative images of the surfaces of rocky worlds that will help you see how they compare in terms of impact craters and volcanoes. Study the images carefully before you read on.
Slide Show 4.57 Study these images of the rocky worlds in our solar system. Do you see any connection between the existence of volcanoes and numbers of impact craters?
You probably noticed a few patterns as you studied the slide show. For example, the Moon and Mercury are both densely cratered, while Mars has a more moderate amount of impact craters and Venus and Earth have relatively few. The following table summarizes key facts about impact craters and volcanoes on the rocky worlds.
World(s) | Distance from Sun (Earth=1) | Diameter (Earth=1) | Active Volcanoes | |
---|---|---|---|---|
Mercury | 0.39 | 0.38 | densely cratered almost everywhere | no |
Venus | 0.72 | 0.95 | relatively few craters | yes |
Earth | 1 | 1 | relatively few craters | yes |
Moon | 1 | 0.27 | densely cratered almost everywhere | no |
Mars | 1.52 | 0.53 | moderately cratered, more in some regions than others | active within the past few hundred million years |
Asteroids (in asteroid belt) | 2 to 3 | < 0.1 | densely cratered almost everywhere | no |
Claim-Evidence-Reasoning Activity
Volcanoes and Craters
Study Slide Show 4.57 and the summary table (above) carefully, then work in small groups to do the following.
- For each world (and asteroids), briefly explain how the images in the slide show support the statements in the table about impact craters and about volcanoes.
- The table states that Mars has volcanoes that have been active within the past few hundred million years, but they are not active today. Based on this fact, scientists usually say that Mars’s volcanoes have been active in “geologically recent” times. Explain how something that happened hundreds of millions of years ago can be considered “geologically recent.” Hint: Remember that the solar system and all the planets are thought to be about 4½ billion years old.
- Based on what you have learned, use evidence and reasoning to support or refute the following claim:
Claim: There is an opposite (inverse) relationship between impact craters and volcanoes: More impact craters means less volcanic activity, and vice versa.
- Now use evidence and reasoning to support or refute each of the following two claims:
Claim 1: A world’s distance from the Sun is a key factor in determining whether it has more impact craters or more volcanoes.
Claim 2: A world’s size (diameter) is a key factor in determining whether it has more impact craters or more volcanoes.
This should be a relatively straightforward activity in which students should end up recognizing that, as we already found earlier for atmospheres, whether or not a world has geological activity (so far we have talked only in terms of volcanoes, but the same ideas also apply to tectonic and erosional activity) depends primarily on its size. Notes:
- (1) For this part, students should make a clear connection between particular images and the statements in the table. E.g., for Mercury, they should point back to images in the slide show as the evidence supporting the statement that Mercury is densely cratered.
- (2) A few hundred million years is “geologically recent” in the sense that it is less than about 10% of a geological time scale that extends back 4½ billion years.
- (3) The claim is true, and the evidence should be clear from study of the table:
- The densely cratered worlds are Mercury, the Moon, and asteroids, and none of these have active volcanoes.
- Venus and Earth are the two worlds with active volcanoes, and both have relatively few impact craters. (Note: “relatively few” is still a decent number; e.g., there are more than 150 identified impact craters on Earth.)
- Mars is the intermediate case that supports the pattern: It has a moderate number of craters, and while it has no active volcanoes today, the fact that it had them in geologically recent times supports the inverse relationship between craters and volcanoes.
- (4) Students should be able to use the table data and what they learned when we asked similar questions about atmospheres. The answers are:
- Claim 1 is false, as should be clear from the lack of any pattern in cratering or volcanoes with distance from the Sun.
- Claim 2 is true, as evidenced from the fact that the small worlds are heavily cratered, the intermediate Mars is moderately cratered, and the large rocky worlds of Venus and Earth have the fewest craters.
Through the above table and activity, you have hopefully discovered that there is an “opposite” (inverse) relationship between craters and volcanoes, in which more volcanoes means fewer impact craters. Moreover, the way in which this relationship plays out on any particular world depends on size in much the same way that size affects atmospheres. In fact, extending our earlier summary for atmospheres (see Figure 4.51), we can now say:
- Small rocky worlds (Mercury, the Moon, and asteroids) have no atmospheres, no active volcanoes, and many impact craters.
- Mars, which is the one and only “medium-size” rocky world in our solar system, has an atmosphere, but not very much of one; volcanoes, but no longer active; and a moderate number of impact craters.
- The two “large” rocky worlds (Venus and Earth) both have substantial atmospheres, active volcanoes, and relatively few impact craters.
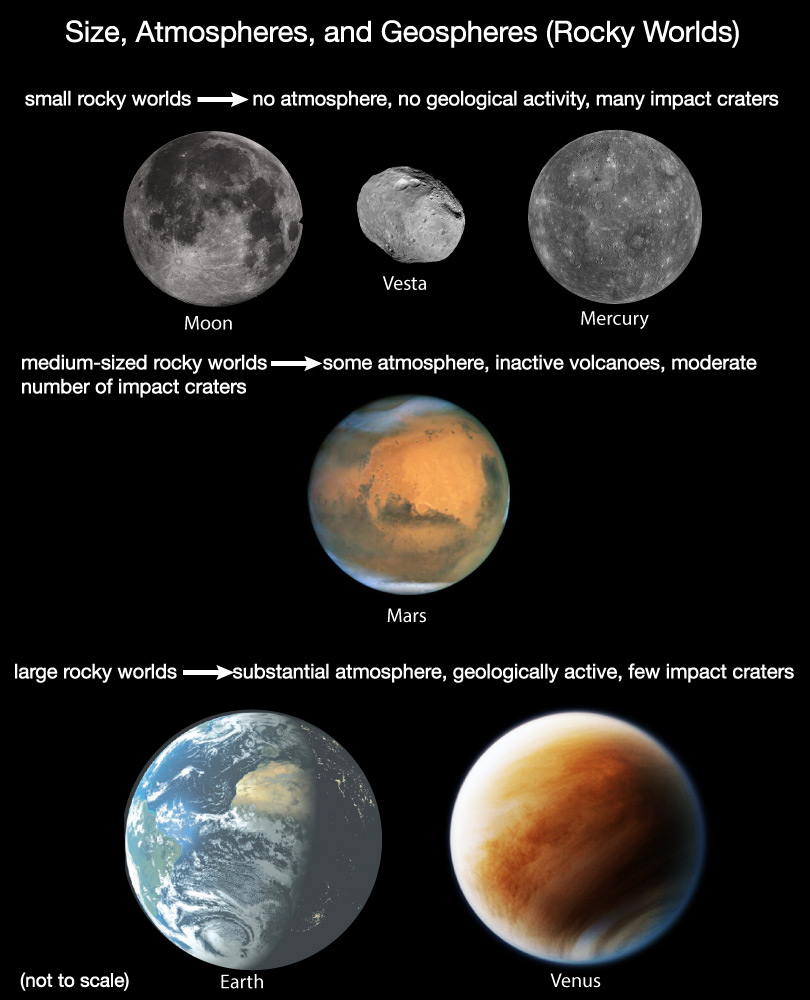
Size and Volcanoes
Recall that the relationship between size and atmosphere is related to the strength of gravity on the different worlds. But why does size also affect craters and volcanoes? Let’s start by considering volcanoes.
Activity (Optional)
Experiments with Internal Heat and Size
Coming Soon
This activity offers two optional experiments that can help your students understand the relationship between internal heat and size while giving practice with data collection and interpretation. It is optional because students are already familiar with the general idea — which is that smaller objects cool more quickly — from their everyday experiences with eating hot foods; they can therefore go straight to the CER activity that follows without first doing this activity. You should therefore decide whether to do this one based on the time you have available (and whether you have the available materials).
Claim-Evidence-Reasoning Activity
Internal Heat and Size
Work in small groups to do the following.
- Discuss, based either on your experiments above or on personal experience: Which cools more quickly, a large hot potato or a small hot potato?
- Suppose you have a very hot potato (or any other very hot food item). If you are hungry but don’t want to burn your tongue, should you just bite into it or cut it up first? Explain why.
- Use evidence and reasoning to support or refute the following claim.
Claim: Smaller objects cool more quickly than larger objects of the same composition.
- Recall that the four rocky planets (Mercury, Venus, Earth, Mars) and the Moon all must once have been very hot inside — hot enough for their interiors to melt and separate by density into core-mantle-crust layers. Based on that fact and what you have learned, divide these five worlds into the following three categories based on how hot you would expect their interiors to be today:
Category 1 = very hot; Category 2 = moderately hot; Category 3 = relatively cool. - Now use evidence and reasoning to support the following claim:
Claim: The reason that larger worlds have more volcanoes is because they retain more internal heat.
This CER activity is designed to help students recognize why size is correlated with volcanic activity. While it is helpful to have first done one of the experiments above, it is not necessary. Notes:
- (1) This first question is very easy, as students should know from experience or from the experiments that the smaller potato will cool more quickly.
- (2) Students should realize that cutting it up makes it cool more quickly, which they should be able to relate back to the first question: Once the pieces are smaller, they cool more quickly just like the smaller potato cools more quickly than the large one.
- (3) They should be able to cite the evidence that answered the first two questions in support of the claim. They can add other similar evidence based on experience with hot objects of different sizes but similar compositions.
- (4) They should now recognize that the amount of internal heat that still remains after 4½ billion years of cooling should correlate with size, so that the two largest worlds (Earth and Venus) would still be very hot inside, Mars is moderately hot, and the smallest worlds (Mercury and the Moon) are relatively cool.
- (5) Students should be able to build an argument to support this claim by citing what they’ve already learned about volcanoes correlating with size, the similar correlation of size and internal heat, and the fact that volcanoes are driven by internal heat.
Through the above activity, you should now understand that larger worlds have more volcanoes because they retain more internal heat. In fact, it’s not just volcanoes that depend on size and internal heat, but geological activity in general. “Geological activity” also includes plate tectonics, erosion , and other size-related processes that that can change a world’s surface appearance. In essence, “geological activity” encompasses all physical processes that can change a world’s surface appearance except for the impacts that make impact craters.
Figure 4.59 summarizes the connection between size, internal heat, and geological activity.
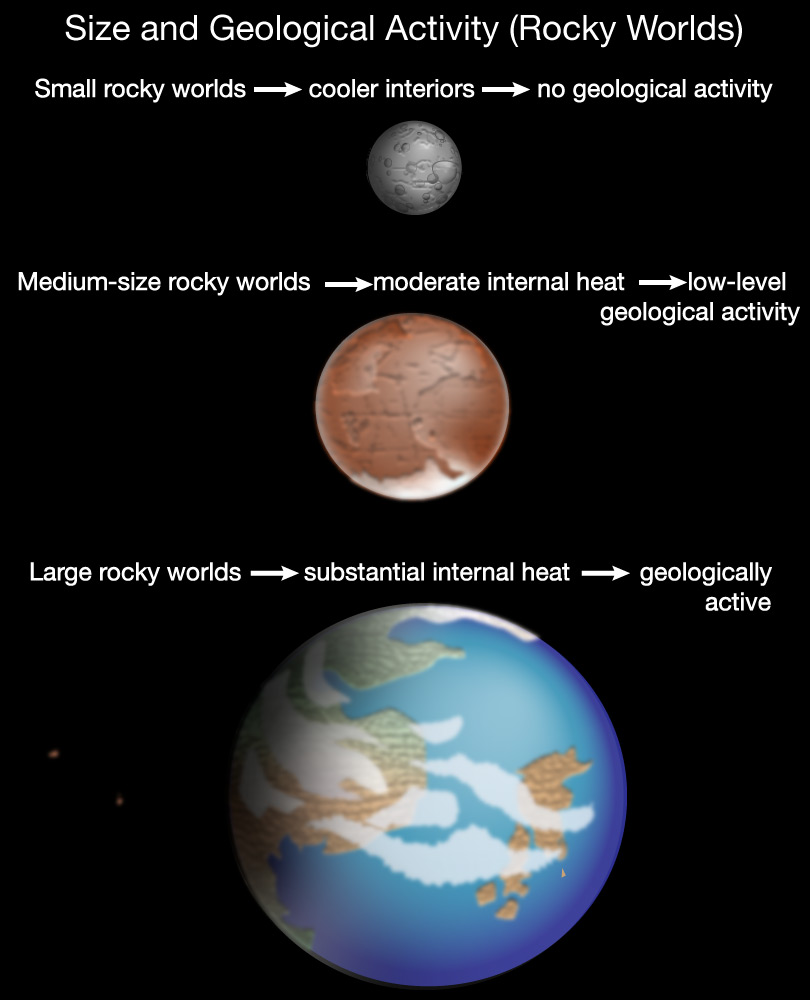
Take It To The Next Level
WHY Do Larger Objects Cool More Slowly?
Coming soon
Impact Craters and Geological Activity
We’ve seen that smaller worlds lack volcanoes and other forms of geological activity because their small sizes mean they don’t have enough internal heat, either because they never had much heat in the first place (small asteroids) or because they’ve cooled too much over time (Mercury, the Moon, and large asteroids like Ceres). But how does this lead to the opposite relationship we’ve seen between impact craters and geological activity? You might already be able to figure out the reason. Try the following questions to see if you are on the right track.
Discuss the following questions with a classmate, then click to open the answers to see if they agree with what you came up with.
- (Multiple Choice) Recall that impact craters are formed when “leftover” objects — asteroids or comets — slam into the surface of a world. Which of the following do you think correctly states when most impacts in the solar system occurred? Explain why.
(a) Most impacts occurred very early in the solar system’s history.
(b) Most impacts have occurred quite recently in the solar system’s history.
(c) Impacts occur evenly through time, so just as many have occurred recently as in the distant past.The answer is (a), for a very simple reason: Every time an asteroid or comet crashes and makes an impact crater, there is one less of them left to cause a crash in the future. In fact, studies of craters on the Moon and models of the solar system’s early history both tell us that the vast majority of impacts occurred within the first few hundred million years of the solar system’s existence. (This is the “period of many large impacts” — sometimes called the heavy bombardment — shown at the beginning of the time line in Figure 4.24.)
- Which of the following two choices do you think is more likely, and why:
(a) All the rocky worlds were hit by many impacts in the distant past.
(b) Only small worlds like Mercury and the Moon were hit by impacts, while larger worlds were immune to impacts.The answer is (a): All worlds were similarly blasted by impacts. The alternative (b) does not make sense, because there is nothing about size that could give any immunity to being hit by asteroids or comets. In fact, large worlds should have been hit by at least as many impacts as smaller worlds, because their larger sizes make them an easier target.
Note: In case you are wondering whether the fact that larger worlds have atmospheres might play a role by causing objects to burn up before they hit the ground: Although small, pebble-size objects burn up as meteors (sometimes called shooting stars) in an atmosphere, the atmosphere has no effect on the larger objects that can make impact craters. You know this in part because you know there are plenty of rock-size meteorites that hit the ground but aren’t large enough to make craters. - Based on your first two answers above, which of the following two choices could explain why a rocky world like Venus or Earth has relatively few impact craters?
(a) It was very lucky not to have been hit by many impacts.
(b) It once had a great many craters, but most of those craters have been buried or erased so that we can no longer see them.The answer is (b). You already know that large worlds should have been hit by at least as many impacts as small worlds, and there’s no chance that “luck” could have prevented that. So the only possible explanation for their lack of craters today is that the craters they had long ago have been buried or erased.
- You know that worlds like Venus and Earth that have few craters have active volcanoes. Could the existence of volcanoes help explain the lack of impact craters? If so, how?
- Recall some of the processes of plate tectonics that we’ve already mentioned, such as how trenches occur where one plate slides under another or how mountains like the Himalayas mark a place where two plates have collided. Briefly explain how these processes could also “erase” impact craters.
These processes can erase impact craters even more effectively than lava flows, because while lava flows only cover a crater over, these processes can completely destroy craters. For example, when one plate slides under another, any impact crater that was previously on the surface gets pulled down underground. Similarly, the plate collision that built the Himalayas would have crumpled and destroyed any craters that had previously been in that location.
We can state the conclusion from the above questions in a simple form, illustrated in Figure 4.60: All rocky worlds must have been covered by impact craters early in the solar system’s history, but on worlds with volcanoes and other geological activity, most or all of those ancient craters have be erased.
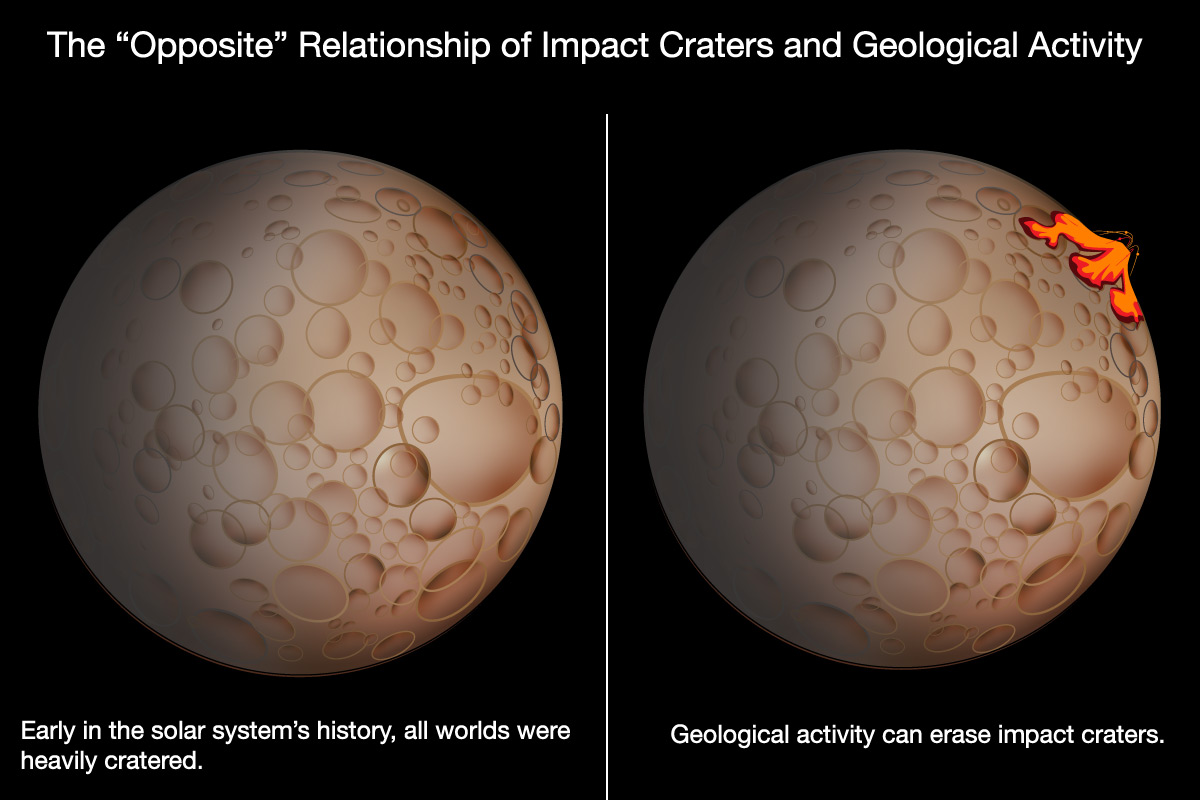
In fact, Venus and Earth are so geologically active that all of their impact craters from early in the solar system’s history are gone. The relatively few impact craters that we see on Venus and Earth today are the result of impacts that have occurred much more recently in geological time.
We have found that a rocky world’s size is the primary factor in determining how much internal heat it has. (Distance from the Sun plays essentially no role in internal heat.) Only the larger rocky worlds retain enough internal heat to drive volcanoes and other geological activity, and the level of geological activity determines how many impact craters we are likely to see on a world’s surface. To summarize (Figure 4.61):
- Small worlds lack geological activity and therefore have surfaces that still show the impact craters made early in the solar system’s history.
- Large worlds still have lots of internal heat, which drives geological activity that has erased ancient impact craters. They therefore have relatively few impact craters, since only relatively recent craters can still be seen.
- Medium-size worlds are the intermediate case, with moderate levels of geological activity and moderate numbers of impact craters.
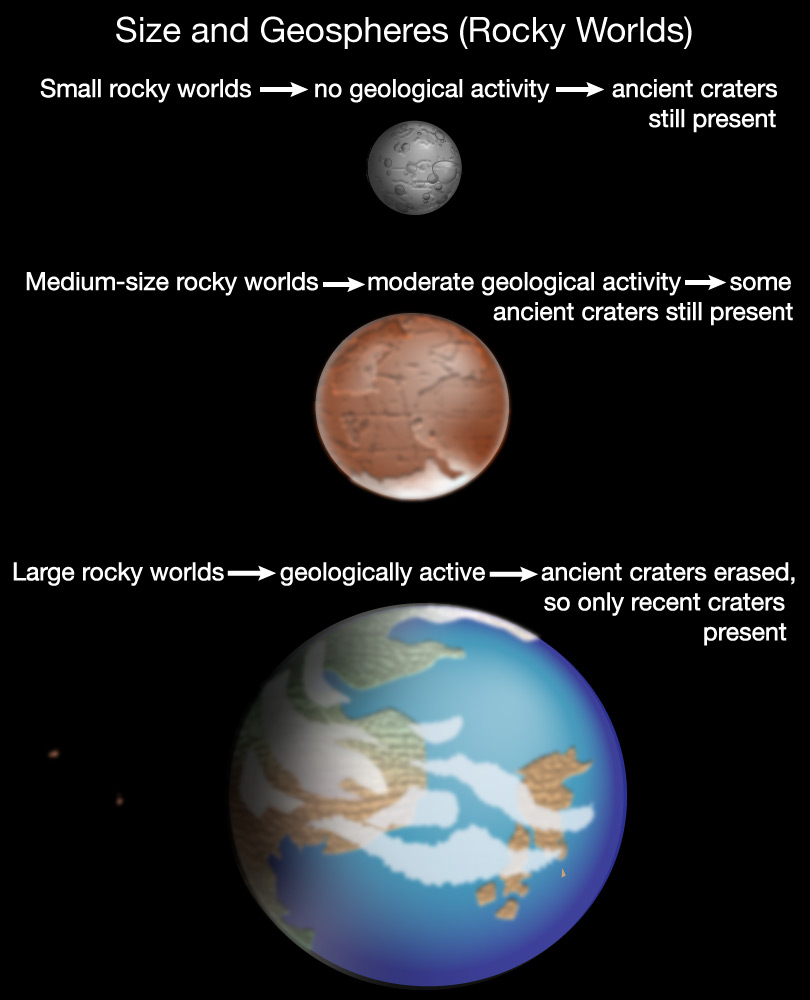
Discussion
Your Future Telescope Project
Scientists have discovered thousands of planets around other stars, which we call extrasolar planets (or exoplanets for short). We don’t yet know much about these exoplanets aside from their sizes and orbits, but scientists hope that future telescopes will be capable of studying them in much more detail.
Imagine that it’s the year 2050, and the global community has just completed building a telescope in space that is larger and more powerful than any previous telescope. Your research team is interested in using the telescope to study exoplanets in search of evidence that could tell us whether they have surface oceans and active volcanoes. However, because there are so many other projects that the telescope can also be used for, you are granted only enough observing time to study one extrasolar planet. Which of the following three planets should you choose to observe, and why?
- Planet 1 is about the same size as Earth and orbits its star beyond the outer edge of the star’s habitable zone.
- Planet 2 is about the same size as Mercury and orbits with the habitable zone of its star.
- Planet 3 is about the same size as Venus and orbits with the habitable zone of its star.
This discussion can be done either in small groups or as a class. The students should be able to settle on Planet 3 as the best choice as follows:
- Planet 1’s size (same as Earth) makes it a good candidate for volcanoes and other geological activity, but the fact that it is outside the habitable zone means it is not a good candidate for oceans.
- Planet 2 is within the habitable zone, but its small size means it is unlikely to have geological activity or to retain the atmosphere that is necessary to having oceans.
- That leaves Planet 3: Being the size of Venus makes it a bit smaller than Earth, but still large enough to have active volcanoes (as Venus does). Being in the habitable zone makes it a good candidate for having oceans.
Using Impact Craters to Estimate Surface Age
This is another optional subsection, with material that goes beyond what is generally expected in middle school science (and is not covered in NGSS for middle school). However, it is not particularly difficult, and the activity should give students some useful insight into an important technique that scientists use in studying other worlds.
Before we continue on, it’s worth noting that the relationships we’ve found provide scientists with an important tool for learning about the geological histories of other worlds.
As you know, scientists have found very strong evidence telling us that all the worlds of our solar system formed at about the same time, 4½ billion years ago. However, the surfaces of these worlds have been modified since that time through impacts, lava flows, and other geological processes. In general, when scientists talk about the “age” of a world’s surface (the surface age ), they mean the time since the surface was last modified in a significant way. Because the vast majority of impacts occurred before the solar system was about 4 billion years old, with only relatively few impacts occurring since that time, only very ancient surfaces are heavily cratered.
Claim-Evidence-Reasoning Activity
Impact Craters and Surface Age
Use all that you have learned so far to argue with evidence and reasoning in support of the following claim.
Claim: If a rocky world has a surface densely covered with impact craters, then the surface has NOT experienced any substantial geological activity (such as volcanoes or plate tectonics) during most of the past 4 billion years.
This CER activity may be challenging because it is a synthesis of so many key ideas, but students should have all they need to make a clear, evidence-based argument. In particular:
- We know that most impacts occurred during the first few hundred million years of the solar system’s history — so this was the period of many impacts (the heavy bombardment).
- The solar system’s age of 4 ½ billion years means that the period of many impacts ended by about or just a little beyond 4 billion years ago.
- Therefore, if a surface is densely covered with impact craters, we can conclude that most of those craters formed before about 4 billion years ago.
- We know that geological activity (volcanoes, plate tectonics) tends to erase craters. Therefore, if old craters have not been erased, there must not have been substantial geological activity.
- In sum, if we see a surface that is densely covered with craters, then we must be seeing it as it was almost 4 billion years ago, which means no substantial geological activity has occurred to erase those craters since that time.
This idea can be generalized to allow scientists to estimate surface ages based on how many and how crowded together impact craters are. If there are very few craters, the surface must have been “repaved” by geological activity fairly recently, so that we only see the recent craters. If there are more craters, then the surface must be older. The details can get technical, but the following activity makes use of the basic ideas.
Activity
Surface Ages
Work in small groups to look at the photos that appear with each question below, using them to answer the question. Remember that by the “age” of a surface, we mean the time since it was last modified by lava flows or other geological processes.
- The photos below show surface regions of the Moon and Mars. Which surface is older, and how do you know?
Surface of Moon. Credit: NASA. Surface of Mars. Credit: NASA. - A single world can have surface regions that differ in their crater counts and hence their ages. For example, the Moon is more heavily cratered in the “lunar highlands” (left photo) than in the smooth maria (right photo). Which region of the Moon’s surface is older, and what must have happened in the maria to explain its smoother appearance? Bonus: Notice that there are still a few craters in the maria; what can you say about when these craters formed?
Lunar highlands. (The "chain" is part of the spacecraft that took the photo.) Credit: NASA. Mare Tranquilitatis. Credit: NASA. - Mars also has surface regions of different ages. The left photo shows a portion of Mars’s “southern highlands.” The right shows the flanks of the volcano Olympus Mons. Which surface region is older, and how does this make sense based on what you see in these photos?
Tagus Valles, southern highlands, Mars. Credit: ESA. Flanks of Olympus Mons, Mars. Credit: ESA. - The same general reasoning we’ve used to understand impact craters on rocky worlds also applies to ice-rich worlds. That is, most craters formed early in the history of the solar system. The photos below show global views of Jupiter’s moons Europa and Callisto. Which moon has the older surface, and how do you know? Bonus: Europa’s surface is made mostly of water ice, and scientists suspect that Europa has an ocean of liquid water buried under this surface. How does the fact that Europa has relatively few impact craters support this idea?
- There is only one world in the solar system that is known to have no impact craters at all: Jupiter’s moon Io, shown in the photo below. The many “pockmarks” visible on Io are active volcanoes — in fact, they are so active that scientists have witnessed many volcanic eruptions on Io. What explains the lack of craters on Io, and what does this tell you about the age of its surface?
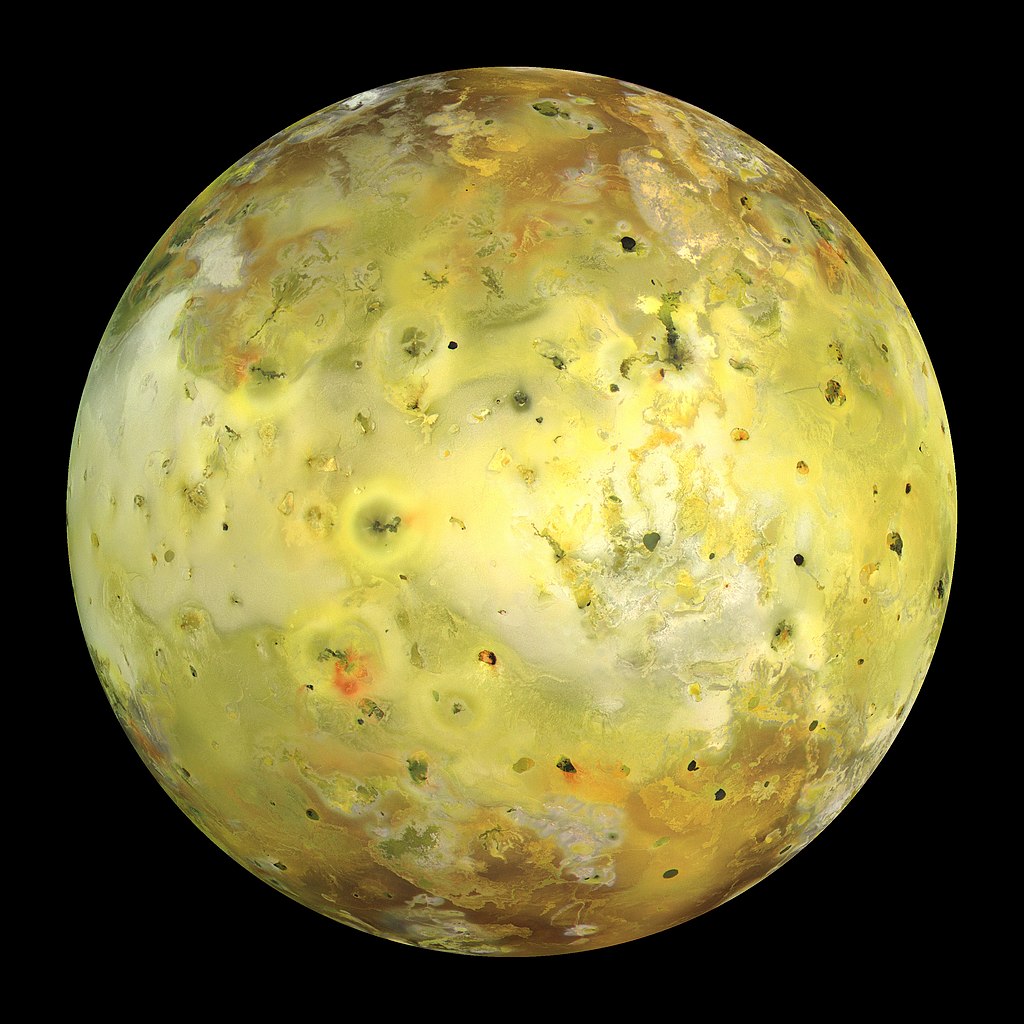
This activity will introduce students to the way in which scientists estimate surface ages from crater crowding. Note that we ask students to do it only qualitatively (e.g., young vs. old), but scientists are able to use these ideas to estimate ages with somewhat more precision, though this method of dating surfaces can still leave uncertainties of many hundreds of millions of years. Notes:
- (1) The Moon’s surface is older because it has more closely crowded craters. The smoother surface of Mars indicates that at least some of the craters that formed during the heavy bombardment have since been erased.
- (2) The lunar highlands are older, looking essentially unchanged from the time of the heavy bombardment ended about 4 billion years ago. The maria must be younger, and their smoothness indicates that the craters that were once in these locations were covered up by lava flows. Bonus answer: The craters found within the maria formed after the lava flows that created the maria. Fyi, while the maria are younger than the highlands, they are still well over 3 billion years old (based on radiometric dating of rocks brought back by the Apollo astronauts.
- (3) The more heavily cratered southern highlands are older. The younger age of the flanks of Olympus Mons make sense because it is a volcano. In fact, it is the relatively small number of craters visible on its flanks that tell scientists that it must have erupted within the past couple hundred million years.
- (4) Callisto’s heavily cratered surface tells us that it is much older than Europa’s lightly cratered surface. This means that Europa’s ancient impact craters have all been erased, and only the craters from relatively recent impacts are visible. Bonus answer: Given that Europa’s surface is made largely of water ice, the most obvious explanation for the erasure of its craters is that water flowed upward from beneath the surface into them. That means there must be enough heating inside Europa to cause these water flows at some times, and the same heating could also cause a layer of subsurface ice to be warm enough to make a subsurface ocean.
- (5) The fact that Io has no craters tells us that its surface must be very young — no more than a few million years old, and likely much younger. The existence of so many volcanoes clearly tells us that it is volcanic eruptions that are erasing its craters. Indeed, Io is by far the most volcanically active world in our solar system.
- For your own understanding: Much of our scientific knowledge of when impacts occurred comes from careful study of craters on the Moon, including radiometric dating of rock samples brought back from the Moon by the Apollo astronauts. These studies not only tell us that most craters formed during the first few hundred million years of the solar system’s history (during the heavy bombardment) but also give us an estimate of the rate of impacts since that time. That is how crater counts can be used to estimate the age of a world’s surface.